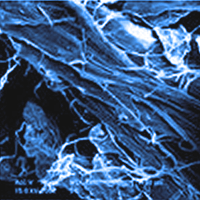
Influence of inorganic salts on biomass production, biochemical composition, and bioethanol production of Populus alba
iForest - Biogeosciences and Forestry, Volume 13, Issue 6, Pages 566-574 (2020)
doi: https://doi.org/10.3832/ifor3438-013
Published: Dec 07, 2020 - Copyright © 2020 SISEF
Research Articles
Abstract
Inorganic salts are very important for the biosynthesis of major components such as cellulose and lignin. In order to investigate biomass production, major components of the biosynthesis of plant cell wall and the bioethanol production of Populus alba, we examined the effect of inorganic salts on in vitro culture systems without specific mineral salts. The medium without H2PO4- was supportive for Populus alba shoot growth, while the absence of NH4+ resulted in poor shoot growth. The medium without H2PO4- and Fe3+ inhibited above-ground biomass production, whereas NH4+ and K+ deprivation led to an enhancement of the same. The root/shoot ratio of Populus alba in the medium without H2PO4- was high compared with plants cultured in the control medium. H2PO4- is deeply involved in lignin biosynthesis, and its removal has been shown to reduce the biosynthesis of lignin. Plants grown on nitrate-free medium were found to be good for enzymatic saccharification and ethanol production. The plants grown in the medium without NO3- showed 72.0% enzyme digestibility, and the yield of ethanol showed 9.58% ethanol productivity after 12 hours. These results can be used as the basis for producing high-quality biomass for future bioethanol production.
Keywords
Enzymatic Hydrolysis, Fermentation, Inorganic Salts, In vitro Culture, Populus alba
Introduction
In vitro plant cultivation provides easy control of environmental factors. The in vitro testing of optimal plant requirements can provide accurate information on cultivation without planting in fields. The preliminary design of the cultivation environment of the plant to be cultivated through in vitro culture methods can be called “in vitro monitoring”. Nutrient media for plant tissue cultures are designed to maintain the plant tissue in a fully artificial environment. In vitro culturing allows the monitoring of plant responses under a variety of biochemical and physiological conditions ([38]).
The short rotation coppice (SRC) is known as an effective way to enhance biomass massive production and reduce atmospheric CO2 accumulation ([2]). SRC energy crops such as poplar (Populus spp.) are grown commercially for heat and power generation as a consequence of their rapid growth rate and favorable energy ratio ([34]). Populus species play significant roles in global ecosystems and provide raw materials for the forest industry and fuels for rural areas in developing countries ([18]). Populus alba (P. alba) is the fastest growing among the Populus species and is easily propagated, and thus it serves as a model for tree biotechnology ([4]).
Crops grown for their high production require nutrients in the soil to be carefully considered. SRC cultivation should also take into account soil nutrients and fertilization ([7]). Several studies of SRC, such as poplar and willow, have studied these nutrients, but they focused on the optimal cultivation environment. There have been no studies on the effect of inorganic salt components on plant cell wall composition.
Nutrient stress can cause a change in the content of lignin and other secondary metabolites in trees ([5]). Tree growth is generally associated with the ability to obtain mineral nutrients from the soil in a balanced amount. The factors that can alter mineral nutrition in plants may also affect the lignification process. Yet, the role of the mineral nutrients in lignification is still unclear. The regulation of nutrient supply in plants may be one of the most easily practicable ways of directing the biosynthesis of lignin and other compounds in plants.
Lignocellulosic biomass can be converted to bioethanol after pretreatment procedures, hydrolysis and subsequent fermentation. The lignocellulosic biomass is mainly composed of cellulose, hemicelluloses and lignin, along with smaller amounts of pectin, protein and ash ([19]). Pretreatment has been viewed as one of the most important and expensive steps in releasing sugars from hemicelluloses and cellulose ([21]). Removing lignin and hemicelluloses, the reduction of cellulose crystallinity and the increase of porosity during the pretreatment processes can significantly improve the enzymatic hydrolysis of cellulose contained in the lignocellulosic material ([39]). Many studies concerning the pretreatment step have been performed, such as the use of liquid hot water, dilute sulfuric acid, alkaline pretreatment ammonia fiber explosion (AFEX) and ammonia recycle percolation (ARP - [16]). However, these pretreatment methods are still inefficient, highly expensive and not environmental-friendly. Recently, ionic liquids (ILs) have been proven to be effective as solvents for lignocellulose pretreatment. This method results in the rapid saccharification of cellulose and hemi cellulose, but it is too expensive, which remains a major obstacle to its commercialization. Therefore, it is very important that the content of lignin in the plant is low and the content of cellulose is high.
Variations in the chemical composition and physical properties of wood depend on plant species and the growth conditions related to their location ([27]), light environment ([32]), temperature ([46]) and the availability of nutrients for trees ([10]).
The chemical and physical properties of the feedstock can affect the choice and efficiency of the biomass conversion process ([44]). The purpose of this study was to evaluate the effects of inorganic salts on the growth of poplar and biosynthesis of major compounds in plants using an in vitro culture system and to investigate the bio-ethanol production efficiency of poplar grown from each inorganic salt.
Materials and methods
Plant materials and preparation of medium with revised inorganic salts
The plant material of P. alba was distributed from the National Institute of Forest Science and planted at the Forestry Education Expansion Center of Gyeongsang National University (South Korea). Two-year-old seedlings were sampled and used for in vitro cultures. In vitro plants were subcultured every 4 weeks in ½ MS medium ([29]) and cultured for 1 year. Plants used in the experiment were cultured for 6 weeks at a height of 5 cm in the early stage of culture. We used a plant with height of 15 cm as the sample for chemical analysis.
The medium was prepared to investigate the effects of inorganic salts on biomass production, the major components of the biosynthesis of plants and cell walls and the bioethanol production of P. alba. An MS medium was used for the in vitro culture of white poplar ([26]). In addition, in a previous study, it was found that ½ MS medium is suitable for culture, and the concentration used in this study was determined within this range.
The ½ MS solid medium was prepared by removing large amounts and trace inorganic salts. T1 is a basic medium composition for MS, and T2 to T8 are treatments in which nitrogen sources (ammonium and nitrate), potassium, calcium, magnesium and iron are removed (Tab. 1). Each treatment contains all of the other ingredients except inorganic salts. The revised medium was adjusted to pH 5.8 after the addition of 0.7% Agar without the addition of growth regulators. The pH of the eight experimental treatments used in this study was 4.3-5.2; therefore, it was neutralized with 1N HCl to reach an optimum pH of 5.8.
Tab. 1 - Mineral salt compositions of media for in vitro culture of P. alba used in this study. T1 (Control, HSM); T2 (HSM without NH4+); T3 (HSM without NO3-); T4 (HSM without H2PO4-); T5 (HSM without K+); T6 (HSM without Ca2+); T7 (HSM without Mg2+); T8 (HSM without Fe3+).
Medium | Mineral salt (mM) | ||||||
---|---|---|---|---|---|---|---|
NH4+ | NO3- | H2PO4- | K+ | Ca2+ | Mg2+ | Fe3+ | |
T1 | 0.515 | 0.985 | 0.031 | 0.503 | 0.748 | 0.751 | 0.010 |
T2 | 0 | 0.985 | 0.031 | 0.503 | 0.748 | 0.751 | 0.010 |
T3 | 0.515 | 0 | 0.031 | 0.503 | 0.748 | 0.751 | 0.010 |
T4 | 0.515 | 0.985 | 0 | 0.503 | 0.748 | 0.751 | 0.010 |
T5 | 0.515 | 0.985 | 0.031 | 0 | 0.748 | 0.751 | 0.010 |
T6 | 0.515 | 0.985 | 0.031 | 0.503 | 0 | 0.751 | 0.010 |
T7 | 0.515 | 0.985 | 0.031 | 0.503 | 0.748 | 0 | 0.010 |
T8 | 0.515 | 0.985 | 0.031 | 0.503 | 0.748 | 0.751 | 0 |
National Renewable Energy Laboratory (NREL Golden, CO, USA) analytical methods for biomass ([30]) were used. Carbohydrates, lignins (acid-insoluble, acid soluble), extractives and the ash content of raw materials were determined according to NREL procedures ([41]). The reagents (H2SO4) used for the analysis of chemical composition, lignin biosynthesis, enzymatic saccharification, ethanol fermentation, etc., were obtained from Sigma-Aldrich (Sigma Aldrich Corp., St. Louis, MO, USA).
In vitro culture in medium revised with inorganic salts
The P. alba stems were surface sterilized, segmented into 2-3 cm pieces and placed on ½ MS solid medium to allow growth. In vitro plantlets were further placed in culture vessels containing 50 mL of ½ MS basal medium containing sucrose (3% w/v). All cultures were maintained under a 16 h light/8 h dark photoperiod in a growth chamber fitted with a cool fluorescent light emitting 25 µmol m-2 s-1 of photosynthetically active radiation (PAR).
To determine variations in biomass by inorganic salt treatments, individual shoots were cultured on the revised ½ MS solid medium without any plant growth regulators for 8 weeks. The effect of inorganic salts on the shoot growth and biosynthesis of major compounds on the plantlets was studied by culturing P. alba on ½ MS medium free from one inorganic salt at a time (Tab. 1).
The shoot growth and growth appearance were recorded after 8 weeks of culture. The number of shoots and leaves, withering rate, and fresh and dry biomass weights were divided into above and below-ground, and the root/shoot rates were calculated according to the measured values.
The leaf chlorophyll content in nutrient-treated plants was measured using an SPAD (Soil Plants Analysis Development)-502® portable chlorophyll meter (Minolta Co., Ltd., Japan). The SPAD values were repeatedly taken at the center of the leaves throughout the experiments.
Lignin biosynthesis assay
For the histochemical analysis of lignin in biomass, the lignin of the cultured biomass was stained with Wiesner reagents ([14]). The poplar shoots were sectioned freehand with a sharp razor blade, and sections were stained with Wiesner reagents. The lignin component was identified as a red color when observed under a light microscope.
Chemical composition of P. alba biomass obtained in different media
To determine the chemical composition of biomass, National Renewable Energy Laboratory analytical methods ([30]) were applied. The shoot tissues (100 g dry weight) were chopped into small pieces and dried at 70 °C for 24 h in vacuo, extracted with ethanol in a Soxhlet extractor for 3 h and dried at 105 °C for 48 h in vacuo. Lignin and holocellulose contents were determined after delignification with NaClO2 ([47]); briefly, a 2.5 g biomass of defatted P. alba was repeatedly (three times) treated with 1 g of NaClO2 in dilute acetic acid solution (0.2 mL) at 70 °C for 1 h. The delignified product, holocellulose, was filtered, washed with distilled water, dried at 105 °C for 48 h in vacuo and weighed.
The α-cellulose content was determined as the insoluble residue in the NaOH (17.5%) aqueous solution. A flask containing a 1 g sample of the holocellulose obtained according to the process above was dissolved in NaOH. The mixture was stirred for 30 min at 20 °C, and 25 mL of distilled water was then added to the mixture. After 5 min, the residue was filtrated, and then supplemented with 40 mL of 10% acetic acid and allowed to stand. The resulting residue was collected by filtration and washed with 1 L of boiling water. The residue that contained α-cellulose was finally dried at 105 °C for 48 h in vacuo and weighed. The contents of lignin, holocellulose, and α-cellulose in the original P. alba chips were calculated as dry weights relative to the original plant mass. The α-cellulose content was quantified as cellulose. The hemicellulose content of the biomass was determined by the subtraction of the α-cellulose from that of holocellulose.
To observe cellulose using field emission scanning electron microscopy (Fe-SEM) analysis, delignificated tissues were air-dried before being used for microscopic examination. The α-cellulose solids were transferred to carbon tape on copper stubs and sputtered with gold (10 mA and 300s) using a high-resolution sputter coater (Agar Scientific Ltd., Stansted, UK). Fe-SEM investigations were carried out with a Philips XL30 S-FEG® Microscope (Netherlands) operated at 15 kV, Magnification 200× or 300×. Images were acquired with Quartz PCI® Version 4 software (Hitachi high-technologies, Japan).
Enzymatic hydrolysis and alcohol fermentation
Enzymatic hydrolysis was carried out on the non-delignified raw material. For enzymatic hydrolysis, 1 g of extractive-free dry biomass was finely ground and transferred to a 250 mL Erlenmeyer flask containing 50 mL of 0.1 M sodium citrate buffer (pH 4.8). Next, appropriate amounts of cellulase (58.56 FPU g-1) and an equal amount of β-glucosidase (24.2 FBG g-1) were added. The flask was placed in a shaking incubator (IS-97IR, Jeio-Tech Co. Korea) and maintained at 50 °C for 36 h (150 rpm). The released glucose in the medium was analyzed with a mutarotase-GOD method employing a glucose CII-test kit (Wako Pure Chemical Industrie Ltd., Osaka, Japan) after the heat-denaturing of enzymes (100 °C for 10 min). The enzymes used for enzymatic hydrolysis were Celluclast® 1.5 L (Novozymes, Bagsvaerd, Denmark), cellulase from Trichoderma reesei and Viscozyme L (Novozymes) as β-glucosidase.
Regarding fermentation, Saccharomyces cerevisiae KCCM 11215 was used for the alcohol fermentation study. The inoculum required for fermentation was developed in YM (Yeast and Mold) liquid medium (yeast extract 3 g L-1, malt extract 3 g L-1, peptone 5 g L-1 and dextrose 10 g L-1). Later, an inoculum (1.5 × 108 cells mL-1) was seeded to fermentation broth (5% v/v).
Ethanol production was quantified by a gas chromatograph (GC - Agilent Technologies, Santa Clara, CA, USA). The fermentation broth obtained after 36 h was filtered through a 0.2 μm filter before GC analysis to remove any coarse particles ([25]) for alcohol estimation. The GC was equipped with an HP-FFAP column of 30 m with an ID 0.25 mm. The detector and injection port were maintained at 200 °C. The combustion gas was a mixture of hydrogen and air, and nitrogen was used as a carrier gas at a flow rate of 40 mL min-1. The quantification of ethanol by the GC was obtained by comparison with a standard curve generated based on the detector response to known amounts of alcohol.
Statistical analysis
A one-way ANOVA was used to test for differences in P. alba growth. Means were separated by Duncan’s multiple range test (α = 0.05). Values were expressed as means ± standard error (SE). All data sets were subjected to analysis with the SPSS® package (SPSS, Inc. Chicago, IL, USA).
Results and discussion
The appearance of shoot growth in medium revised with inorganic salts
The growth of P. alba was influenced by the presence of different inorganic salts in the medium to varying extents (Tab. 2). Major variations were observed with respect to leaf color, leaf width, shoot number and plant height. The leaf color changed to yellow in the absence of K+ in the medium. Poplar stems were fairly well proliferating when cultured in media free from Ca2+. The leaves of poplar were observed to be wider in NH4+ and Mg2+ deficient mediums; however, the removal of NO3- and H2PO4- from growth medium produced narrow plant leaves with the passage of time.
Tab. 2 - Influence of various mineral salts treatment on the shoot growth of P. Alba. Values are mean shoot growth (in cm) of five replicates ± standard error. Different letters indicate significant differences (p<0.05) among populations after Duncan’s multiple range tests. (T1): Control, HSM; (T2): HSM without NH4+; (T3): HSM without NO3-; (T4): HSM without H2PO4-; (T5): HSM without K+; (T6): HSM without Ca2+; (T7): HSM without Mg2+; (T8): HSM without Fe3+; (***): P<0.001; (NS): not significant (P>0.05).
Medium | Culture period (days) | ||||
---|---|---|---|---|---|
1 | 14 | 28 | 42 | 56 | |
T1 | 2.15 ± 0.23a | 2.53 ± 0.33c | 6.18 ± 0.26bc | 8.57 ± 0.44ab | 9.92 ± 0.47a |
T2 | 2.64 ± 0.13a | 4.59 ± 0.36a | 8.77 ± 0.74a | 10.11 ± 0.78a | 11.36 ± 0.23a |
T3 | 2.43 ± 0.28a | 2.87 ± 0.16c | 3.69 ± 0.22d | 3.89 ± 0.23c | 4.19 ± 0.26c |
T4 | 2.71 ± 0.05a | 3.26 ± 0.18bc | 4.12 ± 0.22d | 4.44 ± 0.24c | 4.62 ± 0.29c |
T5 | 2.47 ± 0.19a | 4.18 ± 0.43ab | 8.16 ± 0.62ab | 10.11 ± 0.56a | 10.46 ± 0.63a |
T6 | 2.45 ± 0.19a | 2.76 ± 0.24c | 5.14 ± 0.60cd | 7.01 ± 0.37b | 7.59 ± 0.41b |
T7 | 2.43 ± 0.11a | 3.95 ± 0.28ab | 6.32 ± 0.86bc | 8.86 ± 1.45ab | 9.55 ± 1.38ab |
T8 | 2.59 ± 0.14a | 4.61 ± 0.45a | 7.83 ± 1.00ab | 9.67 ± 1.11a | 10.41 ± 1.04a |
P-value | NS | *** | *** | *** | *** |
The pattern of shoot growth in the presence or absence of various inorganic salts is shown in Tab. 2. The shoot growth pattern was dependent on inorganic salts and their concentrations. The shoot growth rate of P. alba was high when the plant was cultured in medium without NH4+ (mean height = 11.36 cm), while the plantlets cultured in the medium free from NO3- showed poor shoot growth. These results indicate that the ammonium ion has an adverse effect on plant growth. The use of nitrogen sources is different for each plant species. It has been reported that the addition of NH4+ to some plant species causes toxicity and reduced growth. Yan & Xu ([48]) reported that NO3- addition significantly stimulated the growth of above-ground growth (8.4% g-1 N), whereas NH4+ addition showed a greater effect on below-ground growth (5.9% g-1 N).
The plantlets cultured on the medium without H2PO4- showed poor shoot growth. Phosphorus also influenced the growth of P. alba. As phosphorus is an essential macronutrient for plant growth, it limits crop production in many regions of the world ([15]). Gangawar & Parameshwaran ([13]) observed an enhancement in the total photosynthetic area with increasing levels of phosphorus, which ultimately produced higher amounts of dry matter.
The number of stems per individual was different for each medium (Tab. 3). In the medium with a calcium ion removed, the number of stems was very large. The number of leaves was also different for each medium. In the medium where calcium ion was removed, the number of leaves was also overwhelmingly high. There was no significant difference in the number of withered plants. The content of chlorophyll was significantly different among treatments. The treatments with the lowest chlorophyll content were nitrate-free media, and there were no significant differences from the control treatments of all inorganic salts in the media with calcium, magnesium and iron removed.
Tab. 3 - Influence of various mineral salts treatment on the shoot growth of P. Alba. Values are mean shoot growth (in cm) of five replicates ± standard error. Different letters indicate significant differences (p<0.05) among populations after Duncan’s multiple range tests. (T1): Control, HSM; (T2): HSM without NH4+; (T3): HSM without NO3-; (T4): HSM without H2PO4-; (T5): HSM without K+; (T6): HSM without Ca2+; (T7): HSM without Mg2+; (T8): HSM without Fe3+; (***): P<0.001; (NS): not significant (P>0.05).
Treatment | No. of shoots/ explant |
No. of leaves | Withering rate | Chlorophyll |
---|---|---|---|---|
T1 | 1.00 ± 0.00b | 11.25 ± 0.25b | 0.00 ± 0.00a | 33.58 ± 1.75a |
T2 | 1.00 ± 0.00b | 10.40 ± 0.45bc | 0.00 ± 0.00a | 13.80 ± 3.75b |
T3 | 1.00 ± 0.00b | 7.00 ± 0.50c | 0.06 ± 0.27a | 8.50 ± 4.51b |
T4 | 1.00 ± 0.00b | 7.00 ± 0.50c | 0.03 ± 0.22a | 10.23 ± 5.14b |
T5 | 1.00 ± 0.00b | 11.80 ± 0.65b | 0.03 ± 0.22a | 15.55 ± 2.48b |
T6 | 8.20 ± 1.19a | 35.00 ± 3.45a | 0.00 ± 0.00a | 37.03 ± 1.20a |
T7 | 1.40 ± 0.45b | 12.80 ± 0.82b | 0.06 ± 0.55a | 29.55 ± 3.12a |
T8 | 1.00 ± 0.00b | 11.40 ± 0.27b | 0.05 ± 0.67a | 30.15 ± 1.15a |
P-value | *** | *** | NS | *** |
In the calcium-deficient medium, stem growth was reduced, and the stem and leaf numbers increased. This result implies that calcium has a great influence on the growth of P. alba. Parvin et al. ([31]) reported that calcium significantly increased plant height in the growth of each step of tomato. The best results here were found at a concentration of 5 mM of calcium. Calcium is a signaling molecule and acts as a second messenger which is increased in the cytosol by activating the influx channel both in the plasma membrane and tonoplast and plays a significant role in mediating mechanisms involved in the recognition and response to abiotic stresses in plants ([22]).
The variation in biomass production was analyzed by observations with in vitro cultivated shoots after 8 weeks of culture (Tab. 4). The inorganic salt composition of the medium influenced both the above and below-ground biomass production of in vitro cultured P. alba. Biomass production in media with the omission of inorganic salts was enhanced compared to the control. H2PO4- and Fe3+ deprivation inhibited the quantity of above-ground biomass, but NH4+ and K+ limitation enhanced the production of above-ground biomass. On the other hand, the medium without K+ dramatically supported below-ground biomass production. However, other treatments did not influence the production of below-ground biomass. These results indicated that phosphate is an important factor which is responsible for shoot biomass production. Fredeen et al. ([11]) reported that low phosphate has the most striking effect on biomass production, with 85% reduction in total leaf area and 78% reduction in shoot dry weight at low phosphate levels in the medium. Furthermore, Fe3+ is known to affect plant root growth and to improve drought resistance in plants ([40]).
Tab. 4 - Influence of mineral salts on P. alba biomass production. (R/S ratio): root dry weight (g) / shoot dry weight (g); (T1): Control, HSM; (T2): HSM without NH4+; (T3): HSM without NO3-; (T4): HSM without H2PO4-; (T5): HSM without K+; (T6): HSM without Ca2+; (T7): HSM without Mg2+; (T8): HSM without Fe3+; (***): P<0.001; (**): P<0.01; (NS): not significant (P>0.05).
Medium | Fresh Biomass (g plant-1) | Dry Biomass (g plant-1) | R/S ratio |
||
---|---|---|---|---|---|
Aboveground | Underground | Aboveground | Underground | ||
T1 | 0.62 ± 0.01e | 0.09 ± 0.00c | 0.09 ± 0.00d | 0.01 ± 0.00b | 0.09 |
T2 | 1.63 ± 0.06a | 0.17 ± 0.00b | 0.19 ± 0.03a | 0.02 ± 0.00b | 0.09 |
T3 | 0.72 ± 0.01de | 0.16 ± 0.00b | 0.12 ± 0.04bcd | 0.03 ± 0.00b | 0.22 |
T4 | 0.47 ± 0.01f | 0.20 ± 0.00b | 0.10 ± 0.00d | 0.03 ± 0.00b | 0.29 |
T5 | 1.41 ± 0.06b | 0.19 ± 0.00b | 0.17 ± 0.02ab | 0.17 ± 0.00a | 1.00 |
T6 | 1.42 ± 0.06b | 0.35 ± 0.00a | 0.16 ± 0.01abc | 0.04 ± 0.00b | 0.22 |
T7 | 0.88 ± 0.02c | 0.13 ± 0.00b | 0.13 ± 0.00abcd | 0.02 ± 0.00b | 0.12 |
T8 | 0.82 ± 0.01cd | 0.02 ± 0.00c | 0.11 ± 0.01cd | 0.04 ± 0.00b | 0.42 |
P-value | *** | *** | ** | *** | - |
The root/shoot (R/S) ratio of P. alba was variable (0.09-0.29) depending upon the media components. R/S ratio was high (0.29) compared with the control (0.09) following the removal of H2PO4- from media. Also, the R/S ratio was high (1.00) in the medium free from Ca2+.
The root/shoot ratio is usually given as the ratio of the weight of the roots versus the top of a plant. For most trees under normal conditions, the root/shoot ratio is 1:5 to 1:6 ([24], [33]), i.e., the top is 5 to 6 times heavier than the roots ([33]). The difference in the root/shoot ratio in this study is due to the relatively developed roots of the plant cultured in the medium with inorganic salts removed. An increase in soil fertility is commonly associated with a reduction in the root/shoot ratio; that is, shoot growth increases more in terms of weight than root growth ([6]). Several investigators have reported that phosphate is a key element that strongly influences the initiation and growth of cluster roots ([37]).
The chemical composition of P. alba biomass obtained in different media
The chemical compositions of P. alba grown in other media with inorganic salts removed showed significant differences (Fig. 1). Total extract contents varied between P. alba cultivated in media with different inorganic salts. The content of extracts (i.e., secondary metabolites) includes nonstructural components of biomass such as waxes, fats, tannins, sugars, some resins and coloring matter ([20]). The extractive composition of P. alba was 37.21% in the medium free from Ca2+, which was a little higher than that of P. alba biomass produced using control media components. This result indicated that the biosynthesis of nonstructural components such as fat and resin is independent of Ca2+ in P. alba.
Fig. 1 - Chemical composition of P. alba biomass obtained from growth in different media. (T1): Control, HSM; (T2): HSM without NH4+; (T3): HSM without NO3-; (T4): HSM without H2PO4-; (T5): HSM without K+; (T6): HSM without Ca2+; (T7): HSM without Mg2+; (T8): HSM without Fe3+.
Calcium treatment is known to promote plant growth and biosynthesis, as a secondary metabolite of the plant ([1]). Calcium also plays a regulatory role in plant cell metabolism, signal transduction and in the absorption of nutrients across cell membranes ([43]).
Lignin biosynthesis in medium revised with inorganic salts
The lignified tissues of P. alba were visualized with Wiesner (phloroglucinol-HCl) reagents in 8-week-old plantlet stem middle cross-sections (Fig. 2). Although lignin was found in the whole section of P. alba pith, other plants grown under different nutrient conditions showed varying lignin contents from each other. Slightly later during the development of vascular tissue, cambium (ca) became covered with a continuous circumferential band of cells. The lignification appeared to extend throughout the primary medullary or interfascicular ray tissue (data not shown) and was initiated in the phloem fibers (pf) and xylem (x - Fig. 2A-H).
Fig. 2 - Histochemical lignin staining in stem freehand cross-sections from 8 week old P. alba plantlets (white bar: 2 mm). Lignin is stained red. (ca): cambium; (f): interfascicular fiber; (pf): phloem fibers; (pi): pith; (x): xylem. Magnification ×300. (A) T1 (Control, HSM); (B) T2 (HSM without NH4+); (C) T3 (HSM without NO3-); (D) T4 (HSM without H2PO4-); (E) T5 (HSM without K+); (F) T6 (HSM without Ca2+); (G) T7 (HSM without Mg2+) ; (H) T8 (HSM without Fe3+).
Among various media, the nitrogen devoid (Fig. 2B, Fig. 2C) and H2PO4- removal (Fig. 2C) showed differences compared with other treatments. Lignin was noticed in the xylem tissue as well as in surrounding cambial tissues. In particular, the plant tissue in most test conditions showed a deep red color due to the presence of lignin, but the control and H2PO4- free media had less lignin. This result highlights that lignin biosynthesis acts under the strict control of H2PO4- contents. Mineral deficiency has been shown to affect lignin levels in crops ([12]). Phosphorus deficiency has also been reported to increase lignin content ([9]). There is little information on the effect of P supply on lignin biosynthesis, but a lack or excess of inorganic elements such as P may induce the activation of enzymes involved in lignin biosynthesis ([12]).
Pretreated poplar samples could be analyzed for lignin, α-cellulose, and hemicelluloses. The lignin content in P. alba was 24.11% in the control and 24.78% in biomass obtained by culturing in the medium free from H2PO4- (Fig. 1). Poplar hybrids have cellulose contents ranging from 42% to 49%, hemicellulose from 16% to 23% and total lignin contents from 21% to 29% ([35]). In general, the lignin content of P. alba biomass of obtained in inorganic salt-free media was higher than that of the control, except for treatment without H2PO4-. The growth of polar in the medium without H2PO4- leads to a formation of lignin comparable to that in hybrid poplar and in other nutrient conditions. This result indicated that inorganic salts present in the medium influenced lignin biosynthesis. The high concentration of inorganic salts in the medium favors an increased cellulose level in biomass but a decreased lignin level. Entry et al. ([8]) reported that a high N fertility rate decreased the concentration of lignin in longleaf pine (Pinus palustris Mill.) seedlings.
In the treatment without NH4+ (T2), the lignin content was 32.37%, while the treatment without Mg2+ (T7) showed the highest lignin content (33.14% - Fig. 1). Mg deficiency affects plant differentiation, which appears to influence lignin biosynthesis. Huang et al. ([17]) also reported that Mg deficiency affected the differentiation of citrus roots. Phloem impairment in Mg-deficient leaves resulted in the cell wall lignification of both vascular cambium and spongy parenchyma cells. The hemicellulose content of P. alba was found to be 13.22-17.55% of dry weight (Fig. 1). Hemicellulose contents determined under various nutrient conditions were comparable to control plants; however, the hemicellulose content was low (13.22%) in plant material obtained from media without H2PO4-. Thus, inorganic salts slightly influenced the hemicellulose content of P. alba.
The composition of the α-cellulose present in P. alba stem is shown in Fig. 1. The α-cellulose contents of P. alba ranged between 20.74%-33.78%. Almost all cultivated plants contained a higher amount of α-cellulose than hemicellulose. However, the α-cellulose content in the tissue of popular cultured under H2PO4- limitation was found to be higher than others. This observation indicated that H2PO4- is a very important inorganic salt as it favors cellulose biosynthesis in P. alba. It has often been shown that growth is correlated with lignin and cellulose concentration in stems. The cellulose content increases with the radial growth of oak trees and decreases with its height ([3]). Accordingly, intensive lignification may stop the extension of cell walls and cause the growth cessation of plants ([28]). There is a relationship between lignin and cellulose contents; lignin and cellulose biosynthesis in plants depends on several factors related both to the plant and to the environment. Among environmental factors, nutrient exposure has a close relationship with the cellulose level in plants. Tullus et al. ([45]) reported that N, P and K showed a strong relationship with lignin and cellulose levels in the stem wood of hybrid poplar clones.
The structure of α-cellulose purified from P. alba grown under control and different nutrient treatments was characterized by Fe-SEM. The morphology of the leaf sheath fibers and the cellulose microfibrils formed under differential nutrients is revealed in Fig. 3. Most of the lignin and hemicellulose was removed, and the cellulose microfibrils were separated from the original fibers on the completion of chemical treatments. The cellulose structure displayed a regular compact surface structure, and the fibers were arranged in bundles. The cellulose showed a characteristic morphology related to the nutrient growth environment. The cellulosic fiber in the nutrient conditions shown in Fig. 3A, Fig. 3E, Fig. 3F and Fig. 3G showed a flat cellulose surface and did not show microfibril, whereas the cellulose surface shown in Fig. 3B exhibited both cellulose fibers and cellulose, condensed as microfibrils. Depending on the phosphate deficiency, it is necessary to look at the cellulose surface; phosphate deficiency smooths the cellulose surface. Cellulose surface morphology is related to enzyme glycosylation. In the enzymatic saccharification process for bioethanol production, the surface state and specific surface area of the substrate greatly influence the reaction rate of the enzyme with the substrate, which is one of the main factors influencing the saccharification efficiency ([49]). A smooth surface may prevent the enzyme solution from penetrating, thus the saccharification efficiency may be lowered ([23]).
Fig. 3 - Fe-SEM images of α-cellulose from P. alba cultured under different nutrient conditions. (A) T1 (Control, HSM); (B) T2 (HSM without NH4+); (C) T3 (HSM without NO3-); (D) T4 (HSM without H2PO4-); (E) T5 (HSM without K+); (F) T6 (HSM without Ca2+); (G) T7 (HSM without Mg2+) and (H) T8 (HSM without Fe3+).
Enzymatic hydrolysis and alcohol fermentation of biomass
The enzymatic digestibility of the non-delignified raw materials was investigated with Celluclast® 1.5 L and Viscozyme L. The time course of the enzymatic hydrolysis of P. alba is shown in Fig. 4. The saccharification of biomass occurred in direct relation to time. However, the reaction rate remained relatively low after 36 h. The P. alba biomass obtained in the medium free from NO3- was saccharified to 72.0% after 36 h of hydrolysis, which was higher than for biomass produced from the treatment without NH4+ (64%), without H2PO4- (60%) and without Fe3+ (60%). However, the enzymatic digestibility of poplar biomass produced from Ca2+ limited treatment was very low compared to other treatments.
Fig. 4 - Time of enzymatic hydrolysis required for P. alba biomass obtained under different nutrient conditions. (T1): Control, HSM; (T2): HSM without NH4+; (T3): HSM without NO3-; (T4): HSM without H2PO4-; (T5): HSM without K+; (T6): HSM without Ca2+; (T7): HSM without Mg2+; (T8): HSM without Fe3+.
The maximum cellulose yield (33.78%) was obtained at T4 (free from H2PO4-), whereas the medium without NO3- (T3) showed only a 23.28% cellulose yield. However, at 100 g, the saccharification rate was 72% for T3 and 60% for T4, and when converted to sugar yield, T3 was 16.761 g and T4 was 20.268 g. The treatment without H2PO4- had a higher saccharification rate than the medium without NO3- because the cellulose content was high, although the saccharification rate was low.
The enzymatic hydrolysis of the biomass obtained in the phosphate-deficient medium was not high compared to other treatments. The reason for this is shown in Fig. 3. It can be stated that this may be related to the surface shape of cellulose. In other words, it is determined to be the result of the difficulty of the penetration of the enzyme solution due to the smooth cellulose surface. In addition, the difference in the saccharification rate is thought to be due to the impurities contained in the cellulose saccharification solution, the difference in the cellulose structure (length difference) and the saccharification rate. In particular, in the case of T3 and T4, the saccharification rates (72% and 60%, respectively) for 100 g of raw materials (i.e., 28% and 40%, respectively) are due to impurities. In particular, as can be seen from Fig. 1, the content of extractives is significantly higher in T4 than in other treatments. These results also suggest that inorganic salts can affect cellulose composition and therefore saccharification rates.
Lignocellulosic biomass cannot be saccharified by enzymes to high yields without a pretreatment, mainly because the lignin in plant cell walls forms a barrier against enzyme attack ([36]). However, in this study, the enzymatic digestibility of raw materials was relatively high without pretreatments. A high hydrolysis rate was shown without pretreatment. First, it was presumed that the lignin content was low due to the lack of inorganic salt; second, the sample used for analysis was an in vitro plant. That is, it is assumed that the selective mineral limit decreases the lignin content and crystallinity of cellulose and increases the surface area.
Biomass hydrolysate was subjected to the alcoholic fermentation process. The S. cerevisiae KCCM 1215-mediated ethanol production of popular enzymatic hydrolysates is shown in Fig. 5. Ethanol from fermented enzyme hydrolysate was detected at 23 minutes of retention time (Fig. 6). Ethanol production from NO3- limited hydrolysates increased and reached a maximum level after 12 h, yielding 9.58% of ethanol; however, after 24 h, ethanol production decreased. The research have reported conversions (up to 2% w/v ethanol) in 4-6 days ([42]). In this study, ethanol yielded up to 9.58% (w/v) in 12 h from celluloses obtained from media without NO3- and 9.18% (w/v) in 12 h using cellulases derived from biomass obtained from the medium without Ca2+, respectively. The reason for promoting bioethanol production by controlling the nitrogen source is that cell wall constituents are loose. Zhang et al. ([50]) also reported that nitrogen fertilizer decreased cellulosic content and lignin content.
Fig. 5 - Rate of ethanol production from P. alba enzymatic hydrolysates. (M1): Control, HSM; (M2): HSM without NH4+; (M3): HSM without NO3-; (M4): HSM without H2PO4-; (M5): HSM without K+; (M6): HSM without Ca2+; (M7): HSM without Mg2+; (M8): HSM without Fe3+.
Fig. 6 - GC analysis of bioethanol produced by fermentation of enzyme saccharides. (A): ethanol standard; (B): ethanol produced from fermentation.
We have yet to establish the ethanol production conditions for P. alba. Enzymatic saccharification and ethanol fermentation conditions will lead to a very high rate of ethanol productivity of 9.58%. These results indicated that the fermentation time for ethanol production could be shortened with plant cultivation under optimal nutrient conditions.
Inorganic salts showed both negative and positive effects on P. alba growth (Fig. 7). Among inorganic salts, H2PO4- is important for biomass, chemical biosynthesis and bioethanol production. Nutrient regulation may cause the unfastening of tough biomass components and help to accelerate the hydrolysis during pretreatment, which will ultimately reduce the cost of bioethanol production. An understanding of the optimal plant nutritional requirements would allow growers to adjust their fertilization program to soil types. These results can be used as basic data for proper fertilizer controls to yield quality biomass for bioethanol production in the future.
Fig. 7 - Effects of inorganic salts on biomass production, major components biosynthesis of plant and cell wall, and bioethanol production from in vitro cultured P. alba. (P): Positive; (N): Negative.
Conclusion
Our results showed that the regulation of inorganic salts is very important for biomass and bioethanol production. The appropriate use of inorganic salts can change the composition of plant cell walls. In particular, phosphoric acid and nitrogen sources have been shown to be involved in biomass production and cellulosic structure. For biomass production, H2PO4- showed high biomass productivity, but the enzyme saccharification rate was low, so bioethanol production from lignocellulosic biomass was found to be very important for selecting the appropriate inorganic salt. The results of this study could be very useful for the short-term cultivation of feedstock for bioenergy production.
Author Contribution
SJS and SHY contributed equally to this paper (co-first authors).
Acknowledgment
This research was supported by the Basic Science Research Program through the National Research Foundation of Korea (NRF) funded by the Ministry of Education (2017R1D1A1B04036320) and the Forest Resources Management Project of the National Forest Seed and Variety Center.
References
CrossRef | Gscholar
Gscholar
Gscholar
Gscholar
Gscholar
Gscholar
Online | Gscholar
Gscholar
Gscholar
Gscholar
Gscholar
Gscholar
Authors’ Info
Authors’ Affiliation
Hak Gon Kim
Forest Research Department, Gyeongsangnam-do Forest Environment Research Institute, Jinju, 52615 (South Korea)
Eunji Choi 0000-0002-6191-686X
Yuwon Seol 0000-0002-6794-3404
Myung Suk Choi 0000-0003-1464-1573
Division of Environmental Forest Science, Gyeongsang National University, Jinju, 52828 (South Korea)
Hyun Jin Song
Department of Seed and Seedling Management, National Forest Seed Variety Center, Chungju 27495 (South Korea)
Plant Conservation Division, Korea National Arboretum of the Korea Forest Service, Pocheon 11186 (South Korea)
Institute of Agriculture and Life Science, Gyeongsang National University, Jinju, Gyeongnam, 52828 (South Korea)
Corresponding author
Paper Info
Citation
Sim SJ, Yong SH, Park DJ, Choi E, Seol Y, Song HJ, Jeong MJ, Kim HG, Choi MS (2020). Influence of inorganic salts on biomass production, biochemical composition, and bioethanol production of Populus alba. iForest 13: 566-574. - doi: 10.3832/ifor3438-013
Academic Editor
Federica Brunoni
Paper history
Received: Apr 06, 2020
Accepted: Sep 28, 2020
First online: Dec 07, 2020
Publication Date: Dec 31, 2020
Publication Time: 2.33 months
Copyright Information
© SISEF - The Italian Society of Silviculture and Forest Ecology 2020
Open Access
This article is distributed under the terms of the Creative Commons Attribution-Non Commercial 4.0 International (https://creativecommons.org/licenses/by-nc/4.0/), which permits unrestricted use, distribution, and reproduction in any medium, provided you give appropriate credit to the original author(s) and the source, provide a link to the Creative Commons license, and indicate if changes were made.
Web Metrics
Breakdown by View Type
Article Usage
Total Article Views: 34984
(from publication date up to now)
Breakdown by View Type
HTML Page Views: 30076
Abstract Page Views: 2343
PDF Downloads: 1980
Citation/Reference Downloads: 4
XML Downloads: 581
Web Metrics
Days since publication: 1678
Overall contacts: 34984
Avg. contacts per week: 145.94
Article Citations
Article citations are based on data periodically collected from the Clarivate Web of Science web site
(last update: Mar 2025)
Total number of cites (since 2020): 2
Average cites per year: 0.33
Publication Metrics
by Dimensions ©
Articles citing this article
List of the papers citing this article based on CrossRef Cited-by.
Related Contents
iForest Similar Articles
Research Articles
The use of branch enclosures to assess direct and indirect effects of elevated CO2 on photosynthesis, respiration and isoprene emission of Populus alba leaves
vol. 1, pp. 49-54 (online: 28 February 2008)
Research Articles
Conservation of Betula oycoviensis, an endangered rare taxon, using vegetative propagation methods
vol. 13, pp. 107-113 (online: 23 March 2020)
Research Articles
Preliminary study on genetic variation of growth traits and wood properties and superior clones selection of Populus ussuriensis Kom.
vol. 12, pp. 459-466 (online: 29 September 2019)
Research Articles
Verification of new Populus nigra L. clone improvement based on their performance over three rotations
vol. 13, pp. 185-193 (online: 12 May 2020)
Technical Notes
Improving impregnation properties of fir wood to acid copper chromate (ACC) with microwave pre-treatment
vol. 8, pp. 89-94 (online: 01 April 2014)
Research Articles
Improving dimensional stability of Populus cathayana wood by suberin monomers with heat treatment
vol. 14, pp. 313-319 (online: 01 July 2021)
Research Articles
Energy production of poplar clones and their energy use efficiency
vol. 7, pp. 150-155 (online: 23 January 2014)
Research Articles
Thermo-modified native black poplar (Populus nigra L.) wood as an insulation material
vol. 14, pp. 268-273 (online: 29 May 2021)
Research Articles
Kinetic analysis of poplar wood properties by thermal modification in conventional oven
vol. 11, pp. 131-139 (online: 07 February 2018)
Research Articles
Variation of wood and bark density and production in coppiced Eucalyptus globulus trees in a second rotation
vol. 9, pp. 270-275 (online: 08 September 2015)
iForest Database Search
Search By Author
Search By Keyword
Google Scholar Search
Citing Articles
Search By Author
Search By Keywords
PubMed Search
Search By Author
Search By Keyword