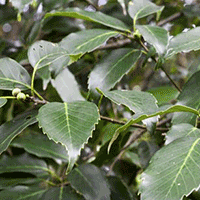
Photosynthesis of three evergreen broad-leaved tree species, Castanopsis sieboldii, Quercus glauca, and Q. myrsinaefolia, under elevated ozone
iForest - Biogeosciences and Forestry, Volume 11, Issue 3, Pages 360-366 (2018)
doi: https://doi.org/10.3832/ifor2493-011
Published: May 04, 2018 - Copyright © 2018 SISEF
Research Articles
Abstract
The main goal of this study was to obtain detailed information on photosynthetic responses of evergreen broad-leaved tree species to ozone (O3). For this, two-year-old seedlings of Castanopsis sieboldii, Quercus glauca, and Q. myrsinaefolia were grown for one growing season, from 15 May to 27 October 2014 under three levels of gas treatments, charcoal-filtered air and 1.0 time and 1.5 times ambient O3 concentrations. We analysed the intercellular CO2 concentration-response curve of the net photosynthetic rate, i.e., the A/Ci curve, in July and October, and growth measurement was carried out at the end of the experiment in October. We observed a difference in O3 susceptibility among the species. Negative effects of O3 were observed on the growth and photosynthetic traits of C. sieboldii, while no significant effects on these traits were noted in the two Quercus species. The decrease in light-saturated net photosynthetic rate (Asat) of C. sieboldii under elevated O3 was accompanied with a significant decrease in the maximum rate of carboxylation (Vcmax). Decreases of leaf nitrogen content and nitrogen use efficiency to Rubisco are considered as factors contributing to lower Vcmax in C. sieboldii seedlings under elevated O3. In addition to the decrease in Vcmax, O3 exposure induced marginal increase of stomatal limitation of photosynthesis. These results indicate that both biochemical and diffusion processes in photosynthesis are responsible for the decrease in Asat of C. sieboldii under elevated O3.
Keywords
Ozone, Photosynthesis, Biochemical Limitation of Photosynthesis, Stomatal Closure, Evergreen Broad-leaved Tree Species
Introduction
Tropospheric ozone (O3) is recognised as a phytotoxic gaseous air pollutant. Many experimental studies have shown the negative effects of O3 on growth and physiological functions such as photosynthesis of tree species ([43], [44], [46]). O3 concentration in East Asia has remarkably increased because of rapid increases in the emissions of main O3 precursors, nitrogen oxides, and volatile organic compounds ([28]). This trend is likely to continue in the near future according to several estimations of gaseous emissions ([24], [47]).
Evergreen broad-leaved trees are the main component species of the laurel forest, which is typical in warm temperate climate zone in East Asia with high amount of precipitation in summer. Japan is located in the northern limits of laurel forest distribution. Castanopsis sieboldii, Quercus glauca, and Q. myrsinaefolia are representative evergreen broad-leaved tree species in the laurel forests of Japan ([25]). These species are also found in urban areas as roadside and park trees in Japan. Ozone sensitivity of evergreen trees is generally considered to be lower than that of deciduous trees ([48], [2], [19]). However, there is a great variation in O3 susceptibility within a functional type. Actually, C. sieboldii is considered as sensitive tree species to O3 and the sensitivity is similar to Fagus crenata, one of the most sensitive tree species to O3 in Japan ([17], [39]).
Photosynthetic carbon assimilation is one of the vital processes essential for the growth and survival of forest trees. The understanding of photosynthetic responses of trees to O3 is crucial to evaluate carbon absorption capacity of a forest under elevated O3. Photosynthetic rate in leaves is regulated by CO2 diffusion from the ambient air to chloroplasts and the biochemical assimilation capacity in chloroplasts ([4], [18]). A distinction between the process of diffusion and the biochemical assimilation by tree species with respect to the response to O3 is useful for a deeper understanding of the effects of O3 on forest production and for the future prediction of carbon sequestration and water balance of forest ecosystems under elevated O3. Kitao et al. ([15]) reported that stomatal limitation of photosynthesis was the main factor behind the decrease in net photosynthetic rate while there was no effect of O3 on the maximum rate of carboxylation (Vcmax), which is in situ activity of Rubisco, in the leaves of mature Fagus sylvatica trees. On the other hand, the opposite results were found in F. crenata saplings ([41]). To our knowledge, there are no current studies that separately evaluate the diffusion and biochemical processes in photosynthesis of East Asian evergreen tree species exposed to O3. Thus, there is a large uncertainty in the risk assessment of the effect of O3 on East Asian laurel forest ecosystems. In the present study, we conducted O3 fumigation study by using three representative evergreen broad-leaved tree species native to Japan, C. sieboldii, Q. glauca, and Q. myrsinaefolia, to evaluate in detail the photosynthetic responses to elevated O3.
Materials and methods
Growth condition, ozone exposure, and plant materials
Greenhouse-type O3-fumigation chambers with natural light source located at the Field Museum (FM) Tamakyuryo of Tokyo University of Agriculture and Technology (35° 04′ N, 139° 02′ E and 144 m a.s.l., Hachioji, Tokyo, Japan) were used in the present study. We set three levels of O3 treatment: charcoal-filtered air (CF, mean O3 removal efficiency: ca. 60%), 1.0 time ambient O3 concentration, and 1.5 times ambient O3 concentration. The ambient O3 concentration outside the chambers was used as the standard for regulating the O3 concentration in the chambers. Three chambers were used for each O3 treatment, with nine chambers in total. Further details of the fumigation and monitoring systems are described in Kinose et al. ([13]).
Two-year-old seedlings of C. sieboldii, Q. glauca, and Q. myrsinaefolia were individually planted in 1/2000 a Wagner’s pots (bulk: 12 L, width: 228-240 mm, depth: 259 mm) filled with brown forest soil (Cambisol according to international classification system - [10]) on 8-9 May 2014. The soil was collected from the A-horizon of the floor of a deciduous forest in the FM Mt. Kusaki of Tokyo University of Agriculture and Technology (36° 03′ N, 139° 02′ E Midori, Gunma, Japan). Before planting the seedlings, the soil was passed through a 5-mm sieve. Emergence of new leaves was observed about one week after planting. Therefore, we considered little plantation shock in the present experiment. On 15 May 2014, the seedlings were transferred into nine chambers and were grown for 166 days until 27 October 2014. In each species, 12 seedlings were assigned to each gas treatment (four seedlings per chamber), with 36 seedlings in total. Mean height and stem base diameter of the seedlings were 39 cm and 3.9 mm for C. sieboldii, 31 cm and 4.8 mm for Q. glauca, and 33 cm and 4.3 mm for Q. myrsinaefolia, respectively. Air temperature and relative air humidity in three of the nine chambers were continuously monitored at 10 min intervals using a TR-72U® Thermo Recorder (T&D Corporation, Nagano, Japan). Light intensity in three of the nine chambers were monitored at 5 min intervals using a HOBO Pendant temperature/light data logger (model UA-002-64®, Onset Computer Co., MA, USA), calibrated against a quantum sensor (model LI-190SA®, Li-Cor Inc., NE, USA).
Daily mean air temperature, relative air humidity, and accumulated photosynthetic proton flux density (PPFD) inside the chambers during the experimental period (15 May to 27 October 2014) were 21.7 °C, 87.0%, and 2881 mol m-2, respectively. The mean light transmissibility of the chambers was approximately 80%. All seedlings were regularly irrigated to maintain soil moisture.
Growth measurement
We measured the height and stem diameter at 2 cm height from the soil surface and leaf number of all seedlings at the end of the experiment (27 October 2014). Leaves that emerged in 2013 and 2014 were separately counted. Stem volume index (square of diameter × height, D2H) was also calculated. We dug out the root of the several seedlings after the experiment to check the root condition in the pot. Although the roots reached the bottom of the pot and circled a little, we did not find intertwining roots. Therefore, we consider the restriction of root growth due to the limited soil volume was little.
Measurement of leaf gas exchange
The gas exchange rates of matured leaves in the upper canopy of the seedlings were determined during 22-30 July and 20-24 October 2014, using an open gas exchange system (LI-6400®, Li-Cor Inc., Lincoln, NE, USA). Two (July) or three (October) seedlings were selected randomly in each chamber (i.e., six or nine seedlings in each gas treatment).
The PPFD during the gas exchange measurement was maintained at 1500 μmol m-2 s-1. Leaf temperature and leaf-to-air vapour pressure deficit were maintained at 28.0 ± 0.5 °C and 1.5 ± 0.2 kPa in July, and 25.0 ± 0.5 °C and 1.5 ± 0.2 kPa in October, respectively. To obtain the intercellular CO2 concentration (Ci)-response curve of A, i.e., A/Ci curve, A was determined at ten CO2 concentration levels in the chamber (Ca - [20]). Firstly, we measured the gas exchange rate under a stable condition of 400 μmol mol-1 of Ca. Then, the Ca was decreased to 60 μmol mol-1 in the following order: 300, 220, 140, and 60 μmol mol-1. After the Ca was increased again to 400 μmol mol-1 and the original A values were confirmed, we increased Ca in the following order: 600, 800, 1100, 1400, and 1700 μmol mol-1. The A, stomatal conductance to water vapour, and ratio of Ci to Ca at Ca = 400 μmol mol-1 CO2 (Asat, Gs, and Ci/Ca, respectively) were calculated from the gas exchange measurement under stable condition (i.e., the first measurement log of A/Ci curve). From the A/Ci curve, we calculated the stomatal limitation of photosynthesis at Ca = 400 μmol mol-1 CO2, the maximum rate of carboxylation (Vcmax), and the maximum rate of electron transport (Jmax - [5], [20]). Values of the Rubisco Michaelis constants for CO2 (Kc) and O2 (Ko), and the CO2 compensation point in the absence of dark respiration (Γ*) for analysis of the A/Ci curve were used according to Bernacchi et al. ([1]). Day respiration was considered as 2% of Vcmax ([36]). The values of Vcmax and Jmax at leaf temperature of 25 °C were calculated using their temperature dependency ([36], [1]). Stomatal limitation (Ls) of photosynthesis was calculated as follows (eqn. 1):
where ACi400 is A at Ci = 400 μmol mol-1 CO2, and ACa400 is A at Ca = 400 μmol mol-1 CO2 (= Asat - [20]).
Measurement of leaf nitrogen content and estimation of leaf nitrogen fraction in photosynthetic functions
After measurement of the gas exchange rate, we collected six leaf discs (diameter, 12 mm each) in order to determine the leaf mass per area (LMA) and nitrogen content. These leaf samples were dried in an oven for 5 days at 80 °C and weighed. The LMA was calculated as leaf dry mass divided by leaf area. The nitrogen content of the leaves per unit mass (Nmass) was determined using a C/N analyser (model MT-700®, Yanaco, Tokyo, Japan). Four of six leaf discs were used for the determination. A calibration curve was generated using hippuric acid (N = 7.82% - Kishida Chemical Co., Ltd., Osaka, Japan). We calculated Narea as the product of LMA and Nmass.
We estimated fraction of leaf nitrogen in Rubisco (Fnr) and bioenergetics (electron carriers except for photosystems, coupling factor, and Calvin cycle enzymes except Rubisco, Fnb). The Fnr was calculated using the following equation ([27], [33] - eqn. 2):
where Vcr is the specific activity of Rubisco (the maximum rate of RuBP carboxylation per unit Rubisco protein), and the factor of 6.25 [g Rubisco (g N in Rubisco)-1] converts nitrogen content to protein content. Here, Vcr is equal to 20.5 μmol CO2 (g Rubisco)-1 s-1 at 25 °C for purified Rubisco enzyme from Spinacia oleracea ([11]). Because this method cannot involve inactivated Rubisco, the calculated value of Fnr is an underestimate ([37]). The Fnb was estimated from gas exchange characteristics according to the following equation ([16], [31] - eqn. 3):
We assumed that the nitrogen in bioenergetics is proportional to Jmax, where the ratio of Jmax to the cytochrome f content is 156 mmol mol-1 s-1 ([26]), and the nitrogen in bioenergetics per unit cytochrome f is 9.53 mol mmol-1 ([6]).
Statistical analysis
Statistical analyses were undertaken using R software, version 3.4.0 ([30]). To test the effects of O3 on leaf and growth parameters, we applied a generalized linear mixed model with average ozone concentration as a fixed-effect and difference of chamber as a random-effect. Log-transformation was applied to growth parameter before the analysis. Because we confirmed the normality for all parameters by Shapiro-Wilk test, response variables were assumed to follow Gaussian distribution in the model.
Results
The average concentrations of O3 for 24 h and daylight hours during the experimental period were 10.7 and 13.0 nmol mol-1 in CF treatment, 19.2 and 24.3 nmol mol-1 in 1.0 time ambient O3 treatment, and 27.6 and 35.8 nmol mol-1 in 1.5 times ambient O3 treatment, respectively (Tab. 1). Daylight AOT40 (accumulated O3 exposure over a threshold of 40 nmol mol-1 with solar radiation > 50 W m-2) in CF, 1.0 time, and 1.5 times ambient O3 treatments during the experimental period were 0.1, 4.1, and 14.1 μmol mol-1 h, respectively.
Tab. 1 - Average concentration and accumulated exposure over a threshold of 40 nmol mol-1 (AOT40) of ozone in each gas treatment during the experimental period for 166 days from 15 may to 27 October 2014. Each value is the mean of three chamber replicates, and the standard error is shown in parenthesis. (Daylight hours): solar radiation > 50 W m-2.
Treatment | Concentration (nmol mol-1) |
AOT40 (μmol mol-1 h) Daylight hours |
|
---|---|---|---|
24 hour | Daylight hours |
||
CF | 10.7 ± 0.6 | 13.0 ± 0.8 | 0.1 ± 0.0 |
1.0 × O3 | 19.2 ± 0.4 | 24.3 ± 0.2 | 4.1 ± 0.0 |
1.5 × O3 | 27.6 ± 0.2 | 35.8 ± 0.2 | 14.1 ± 0.3 |
Height and D2H of C. sieboldii seedlings was significantly decreased by exposure to O3, while stem diameter remained unaltered (Tab. 2). There was a tendency of O3-induced decrease in the number of 2013 leaves. We did not observe significant effects of O3 on any growth parameters examined in Q. glauca and Q. myrsinaefolia seedlings.
Tab. 2 - Height, stem diameter, stem volume index (square of diameter × height, D2H) and leaf number emerged in 2013 and 2014 of Castanopsis sieboldii, Quercus glauca and Q. myrsinaefolia seedlings grown under three levels of ozone fumigation at the end of the experiment. Values are means ± standard error (n = 12). (CF): charcoal-filtered air; (1.0 × O3): 1.0 time ambient ozone concentration; (1.0 × O3): 1.5 times ambient ozone concentration. (*): P < 0.05; (ns): not significant. The actual P-values are shown when 0.05 < P < 0.10.
Species | Treament | Height (cm) |
Stem diameter (mm) |
D2H (cm3) |
Leaf number | |
---|---|---|---|---|---|---|
2013 leaves | 2014 leaves | |||||
C. sieboldii | CF | 124.7 ± 3.3 | 12.7 ± 0.2 | 205.3 ± 9.8 | 9.1 ± 1.0 | 200.8 ± 10.8 |
1.0 × O3 | 115.5 ± 3.9 | 12.7 ± 0.4 | 191.2 ± 13.8 | 7.4 ± 1.2 | 195.4 ± 11.8 | |
1.5 × O3 | 108.1 ± 2.7 | 12.2 ± 0.2 | 165.3 ± 8.6 | 6.4 ± 0.9 | 179.3 ± 10.5 | |
Sig. | * | ns | * | 0.064 | ns | |
Q. glauca | CF | 86.6 ± 3.9 | 11.3 ± 0.3 | 115.3 ± 10.7 | 24.9 ± 1.8 | 82.9 ± 9.8 |
1.0 × O3 | 94.6 ± 3.0 | 11.0 ± 0.2 | 115.8 ± 6.6 | 22.4 ± 2.3 | 94.8 ± 7.0 | |
1.5 × O3 | 93.3 ± 2.6 | 11.2 ± 0.2 | 117.0 ± 5.1 | 22.8 ± 1.5 | 90.4 ± 7.4 | |
Sig. | ns | ns | ns | ns | ns | |
Q. myrsinaefolia | CF | 95.1 ± 4.0 | 11.8 ± 0.4 | 136.9 ± 14.8 | 21.3 ± 2.2 | 83.6 ± 7.3 |
1.0 × O3 | 95.0 ± 2.9 | 11.9 ± 0.3 | 135.7 ± 6.7 | 21.1 ± 2.1 | 104.0 ± 10.0 | |
1.5 × O3 | 97.2 ± 2.9 | 11.7 ± 0.3 | 133.5 ± 9.0 | 22.3 ± 2.2 | 104.3 ± 12.5 | |
Sig. | ns | ns | ns | ns | ns |
There were no significant effects of O3 on gas exchange parameters of all tree species in July, although the Asat of Q. myrsinaefolia showed a tendency to increase after exposure to O3 (Tab. 3). In October, we observed significant O3-induced decreases in Asat and Vcmax of C. sieboldii seedlings (Tab. 4). In addition, there was a tendency of increase in Ls of photosynthesis with increasing exposure to O3. The exposure to O3 did not affect any gas exchange parameters of Q. glauca and Q. myrsinaefolia seedlings in October. We found no significant effects of O3 on LMA, leaf nitrogen content and leaf nitrogen fraction in photosynthetic functions in any of the tree species studied in July and October, except for Nmass of Q. myrsinaefolia in July and Narea of C. sieboldii in October (Tab. 5 and Tab. 6). There were tendencies of O3-induced increase in Nmass of Q. myrsinaefolia in July and decrease in Narea of C. sieboldii in October.
Tab. 3 - Photosynthetic traits of Castanopsis sieboldii, Quercus glauca and Q. myrsinaefolia seedlings grown under three levels of ozone fumigation in July 2014. Values are means ± standard error (n = 6). Abbreviations of experimental treatments are shown in Tab. 2. (Asat): light-saturated net photosynthetic rate (μmol m-2 s-1); (Gs): stomatal conductance to water vapor (mol m-2 s-1); (Ci/Ca): ratio of intercellular CO2 concentration to ambient CO2 concentration; (Ls): stomatal limitation of photosynthesis; (Vcmax): maximum rate of carboxylation (μmol m-2 s-1); (Jmax): maximum rate of electron transport (μmol m-2 s-1); (ns): not significant. The actual P-values are shown when 0.05 < P < 0.10.
Species | Treatment | A sat | G s | Ci/Ca | L s | V cmax | J max |
---|---|---|---|---|---|---|---|
C. sieboldii | CF | 16.0 ± 0.6 | 0.23 ± 0.02 | 0.63 ± 0.03 | 0.23 ± 0.02 | 73.4 ± 6.1 | 98.8 ± 3.7 |
1.0 × O3 | 14.2 ± 0.4 | 0.19 ± 0.02 | 0.62 ± 0.02 | 0.27 ± 0.02 | 62.3 ± 3.0 | 93.8 ± 5.6 | |
1.5 × O3 | 14.8 ± 1.3 | 0.20 ± 0.02 | 0.62 ± 0.02 | 0.26 ± 0.02 | 65.7 ± 7.4 | 97.0 ± 7.7 | |
Sig. | ns | ns | ns | ns | ns | ns | |
Q. glauca | CF | 12.7 ± 1.4 | 0.13 ± 0.02 | 0.56 ± 0.03 | 0.36 ± 0.04 | 81.1 ± 11.1 | 112.8 ± 8.9 |
1.0 × O3 | 11.4 ± 0.8 | 0.13 ± 0.02 | 0.57 ± 0.03 | 0.30 ± 0.04 | 68.5 ± 1.6 | 96.7 ± 4.3 | |
1.5 × O3 | 12.6 ± 0.5 | 0.15 ± 0.02 | 0.61 ± 0.02 | 0.30 ± 0.03 | 73.1 ± 3.0 | 104.6 ± 6.1 | |
Sig. | ns | ns | ns | ns | ns | ns | |
Q. myrsinaefolia | CF | 12.2 ± 0.4 | 0.18 ± 0.01 | 0.63 ± 0.01 | 0.25 ± 0.01 | 62.4 ± 1.7 | 93.7 ± 3.4 |
1.0 × O3 | 12.7 ± 0.3 | 0.16 ± 0.01 | 0.63 ± 0.01 | 0.26 ± 0.01 | 67.2 ± 2.2 | 100.0 ± 2.5 | |
1.5 × O3 | 13.1 ± 0.4 | 0.18 ± 0.02 | 0.65 ± 0.02 | 0.24 ± 0.03 | 67.7 ± 3.3 | 101.1 ± 5.9 | |
Sig. | 0.083 | ns | ns | ns | ns | ns |
Tab. 4 - Photosynthetic traits of Castanopsis sieboldii, Quercus glauca and Q. myrsinaefolia seedlings grown under three levels of ozone fumigation in October 2014. Values are means ± standard error (n = 9). Abbreviations of experimental treatments and photosynthetic parameters are shown in Tab. 2 and Tab. 3 respectively. (*): P < 0.05; (ns): not significant. The actual P-values are shown when 0.05 < P < 0.10.
Species | Treatment | A sat | G s | Ci/Ca | L s | V cmax | J max |
---|---|---|---|---|---|---|---|
C. sieboldii | CF | 13.8 ± 0.8 | 0.17 ± 0.01 | 0.63 ± 0.01 | 0.24 ± 0.02 | 74.3 ± 4.5 | 126.7 ± 9.9 |
1.0 × O3 | 14.1 ± 0.5 | 0.17 ± 0.01 | 0.63 ± 0.01 | 0.25 ± 0.02 | 72.7 ± 2.5 | 126.2 ± 5.2 | |
1.5 × O3 | 11.9 ± 0.4 | 0.14 ± 0.01 | 0.62 ± 0.02 | 0.30 ± 0.03 | 61.7 ± 2.5 | 113.7 ± 5.1 | |
Sig. | * | ns | ns | 0.094 | ** | ns | |
Q. glauca | CF | 15.1 ± 0.5 | 0.18 ± 0.01 | 0.62 ± 0.01 | 0.36 ± 0.01 | 79.9 ± 2.2 | 163.2 ± 4.9 |
1.0 × O3 | 15.4 ± 0.7 | 0.19 ± 0.01 | 0.64 ± 0.01 | 0.33 ± 0.02 | 79.4 ± 3.4 | 155.7 ± 4.5 | |
1.5 × O3 | 15.4 ± 0.6 | 0.20 ± 0.01 | 0.65 ± 0.02 | 0.34 ± 0.01 | 78.6 ± 2.4 | 160.2 ± 5.8 | |
Sig. | ns | ns | ns | ns | ns | ns | |
Q. myrsinaefolia | CF | 14.1 ± 0.6 | 0.16 ± 0.01 | 0.62 ± 0.02 | 0.35 ± 0.02 | 76.6 ± 5.4 | 156.6 ± 10.2 |
1.0 × O3 | 13.2 ± 0.8 | 0.15 ± 0.01 | 0.60 ± 0.02 | 0.35 ± 0.02 | 71.4 ± 3.6 | 140.7 ± 7.7 | |
1.5 × O3 | 13.3 ± 0.4 | 0.15 ± 0.01 | 0.61 ± 0.02 | 0.36 ± 0.02 | 70.9 ± 3.4 | 140.9 ± 6.3 | |
Sig. | ns | ns | ns | ns | ns | ns |
Tab. 5 - Leaf mass per area (LMA, g m-2), mass- and area-based nitrogen content (Nmass and Narea, % and g m-2, respectively) and fraction of leaf nitrogen in Rubisco (Fnr) and bioenergetics (electron carriers except for photosystems, coupling factor and Calvin cycle enzymes except Rubisco, Fnb) of Castanopsis sieboldii, Quercus glauca and Q. myrsinaefolia seedlings grown under three levels of ozone fumigation in July 2014. Values are means ± standard error (n = 6). Abbreviations of experimental treatments are shown in Tab. 2. (ns): not significant. The actual P-values are shown when 0.05 < P < 0.10.
Species | Treatment | LMA | N mass | N area | F nr | F nb |
---|---|---|---|---|---|---|
C. sieboldii | CF | 80.2 ± 1.7 | 2.34 ± 0.07 | 1.88 ± 0.08 | 0.301 ± 0.022 | 0.036 ± 0.001 |
1.0 × O3 | 75.7 ± 1.8 | 2.42 ± 0.07 | 1.83 ± 0.04 | 0.264 ± 0.013 | 0.034 ± 0.002 | |
1.5 × O3 | 76.5 ± 2.9 | 2.34 ± 0.04 | 1.79 ± 0.09 | 0.281 ± 0.019 | 0.036 ± 0.002 | |
Sig. | ns | ns | ns | ns | ns | |
Q. glauca | CF | 95.5 ± 3.7 | 2.21 ± 0.10 | 2.10 ± 0.05 | 0.298 ± 0.038 | 0.036 ± 0.003 |
1.0 × O3 | 90.5 ± 2.3 | 2.20 ± 0.05 | 1.99 ± 0.07 | 0.268 ± 0.010 | 0.033 ± 0.002 | |
1.5 × O3 | 97.4 ± 2.2 | 2.26 ± 0.04 | 2.19 ± 0.04 | 0.258 ± 0.011 | 0.032 ± 0.002 | |
Sig. | ns | ns | ns | ns | ns | |
Q. myrsinaefolia | CF | 88.7 ± 2.2 | 2.02 ± 0.03 | 1.79 ± 0.07 | 0.271 ± 0.007 | 0.035 ± 0.001 |
1.0 × O3 | 87.0 ± 2.5 | 2.07 ± 0.05 | 1.80 ± 0.03 | 0.290 ± 0.011 | 0.037 ± 0.001 | |
1.5 × O3 | 85.1 ± 1.5 | 2.12 ± 0.04 | 1.80 ± 0.02 | 0.290 ± 0.012 | 0.038 ± 0.002 | |
Sig. | ns | 0.081 | ns | ns | ns |
Tab. 6 - Leaf mass per area (LMA, g m-2), mass- and area-based nitrogen content (Nmass and Narea, % and g m-2, respectively) and fraction of leaf nitrogen in Rubisco (Fnr) and bioenergetics (electron carriers except for photosystems, coupling factor and Calvin cycle enzymes except Rubisco, Fnb) of Castanopsis sieboldii, Quercus glauca and Q. myrsinaefolia seedlings grown under three levels of ozone fumigation in October 2014. Values are means ± standard error (n = 9). Abbreviations of experimental treatments are shown in Tab. 2. (ns): not significant. The actual P-values are shown when 0.05 < P < 0.10.
Species | Treatment | LMA | N mass | N area | F nr | F nb |
---|---|---|---|---|---|---|
C. sieboldii | CF | 94.6 ± 1.6 | 2.13 ± 0.09 | 2.00 ± 0.06 | 0.288 ± 0.015 | 0.043 ± 0.003 |
1.0 × O3 | 92.5 ± 2.1 | 2.08 ± 0.07 | 1.92 ± 0.07 | 0.296 ± 0.015 | 0.045 ± 0.002 | |
1.5 × O3 | 88.8 ± 2.8 | 2.08 ± 0.09 | 1.83 ± 0.05 | 0.260 ± 0.006 | 0.042 ± 0.002 | |
Sig. | ns | ns | 0.063 | ns | ns | |
Q. glauca | CF | 97.3 ± 1.6 | 2.58 ± 0.05 | 2.51 ± 0.04 | 0.247 ± 0.008 | 0.044 ± 0.001 |
1.0 × O3 | 97.0 ± 2.6 | 2.58 ± 0.04 | 2.50 ± 0.08 | 0.246 ± 0.008 | 0.042 ± 0.001 | |
1.5 × O3 | 99.1 ± 2.0 | 2.60 ± 0.06 | 2.57 ± 0.07 | 0.237 ± 0.008 | 0.042 ± 0.001 | |
Sig. | ns | ns | ns | ns | ns | |
Q. myrsinaefolia | CF | 92.8 ± 1.8 | 2.54 ± 0.14 | 2.36 ± 0.14 | 0.251 ± 0.008 | 0.045 ± 0.002 |
1.0 × O3 | 89.6 ± 2.3 | 2.62 ± 0.08 | 2.34 ± 0.08 | 0.236 ± 0.009 | 0.040 ± 0.001 | |
1.5 × O3 | 88.8 ± 2.6 | 2.49 ± 0.05 | 2.22 ± 0.09 | 0.248 ± 0.010 | 0.043 ± 0.002 | |
Sig. | ns | ns | ns | ns | ns |
Discussion
We observed a difference in O3 susceptibility among the three tree species. According to the photosynthetic and growth responses to O3, C. sieboldii showed higher susceptibility than the other two Quercus species. The higher susceptibility of C. sieboldii to O3 is in agreement with the results of our previous studies ([17], [39]).
The difference of O3 uptake into the leaves through the stomata is considered an important factor in determining the different susceptibilities to O3 among the tree species ([12]). Stomatal O3 uptake rate can be explained by stomatal conductance to O3 (GO3) when the meteorological condition and atmospheric O3 concentration are the same. The GO3 is proportional to Gs with a proportionality constant of 0.663 (i.e., GO3 = 0.663 Gs - [23]). Thus, the difference of Gs among tree species is considered a good indicator of the difference of stomatal O3 uptake. However, there was no great difference between Gs of C. sieboldii and that of the other two Quercus species (Tab. 3 and Tab. 4), indicating that the amount of O3 uptake would not be largely different among these trees and would not serve as the main parameter to evaluate the difference in O3 susceptibility.
Some Quercus species emit biogenic volatile organic compounds (BVOCs), mainly isoprene, and the BVOC may act as a detoxifying substance for O3 and reactive oxygen species ([21]). However, the BVOC emission varied among the Quercus species. According to Tani & Kawawata ([32]), Q. glauca and Q. myrsinaefolia, which are tolerant species to O3 in the present study, do not emit BVOC. Li et al. ([19]) suggested that LMA is a potentially useful indicator for evaluating O3 susceptibility based on an analysis using 29 tree species, both deciduous and evergreen. In the present study, however, we observed quite similar values of LMA for the three species. Although the reasons for the different O3 susceptibilities between C. sieboldii and the two Quercus species are not clear, it is clear that not all evergreen broad-leaved tree species are tolerant to O3 ([48], [2], [19]). Further screening studies must be conducted to find other “O3-sensitive” evergreen broad-leaved tree species, especially in the genus Castanopsis.
The decrease in Asat of C. sieboldii was accompanied by a significant decrease in Vcmax, marginal decrease in Gs, and increase in Ls (Tab. 4). This indicates that both biochemical and diffusion processes in photosynthesis are responsible for the decrease in Asat under elevated O3, although the contribution of the former may be higher to some degree. O3-induced decrease in Vcmax was reported in several Japanese deciduous tree species ([8], [9], [41], [42]). The Vcmax represents in situ activity of Rubisco ([5], [18]), and a decrease in Rubisco content under elevated O3 was also reported in deciduous trees ([45], [38]).
In contrast to Vcmax, O3-induced decrease of Jmax in C. sieboldii seedlings was not observed (Tab. 4), although decreases in both Vcmax and Jmax under elevated O3 were reported in several studies of deciduous trees native to Japan ([7], [9], [41]). Kinose et al. ([14]) indicated that the timing of significant O3-induced decrease in Vcmax was earlier than that in Jmax of F. crenata seedlings. There is a possibility that the first effect of O3 on the biochemical process of photosynthesis occurs in carboxylation by Rubisco.
Nitrogen is a nutrient that is strongly related to the biochemical assimilation capacity of plants ([18]). A large fraction of nitrogen in the leaves is incorporated into proteins associated with the photosynthetic process ([3]), and the biggest destination of nitrogen investment is Rubisco ([27], [31], [40]). In the present study, we observed marginally significant decrease in Narea and no significant decrease in Fnr of C. sieboldii seedlings under elevated O3. However, the reduction rates of Narea and Fnr are similar, 8.5% and 9.7%, respectively, in 1.5 times ambient O3 treatment compared to CF treatment. Therefore, we consider both factors (i.e., nitrogen content and nitrogen use efficiency to Rubisco) contribute to the decrease in Vcmax under elevated O3
We observed elevated O3-induced increase of Ls in C. sieboldii seedlings (Tab. 4). A similar result was observed in mature F. sylvatica ([15]), but it is in contrast with the results of many other studies of deciduous tree species. Hoshika et al. ([8]) reported the avoidance of O3 uptake by stomatal closure in F. crenata saplings in early summer, but not in late summer and autumn. Plant physiological studies have shown several mechanisms of O3-induced stomatal closure such as direct modulation of K+ channels ([34], [35]), change of Ca2+ homeostasis in guard cells ([22]), and production of phytohormones ([29]).
Conclusions
To our knowledge, this is the first study to analyse photosynthesis with respect to diffusion and biochemical processes in the leaves of East Asian evergreen tree species under elevated O3. High O3 susceptibilities on photosynthesis and growth in C. sieboldii were confirmed. On the other hand, the two Quercus species showed high tolerance against O3. The negative effect of O3 on net photosynthesis of C. sieboldii was due to decreases in both diffusion (increase in Ls) and biochemical processes (decrease in Vcmax). These results indicate that not only the decrease in carbon absorption capacity but also the change in water balance due to stomatal closure should be considered as risks for C. sieboldii forest under elevated O3.
Acknowledgments
The authors are greatly indebted to Mr. Yoshinobu Fukamachi, Ms. Hiroka Hiroshima, and Mr. Shigeaki Okabe (Tokyo University of Agriculture and Technology) for their technical support, and to Dr. Hayato Iijima for the advice of statistical analysis. This study was supported by JSPS KAKENHI, Young Scientists B (15K16136 to MW), and Challenging Exploratory Research (15K12217 to TI and MW). We would like to thank Editage (⇒ http://www.editage.jp/) for English language editing.
References
CrossRef | Gscholar
CrossRef | Gscholar
Gscholar
CrossRef | Gscholar
Online | Gscholar
Gscholar
CrossRef | Gscholar
CrossRef | Gscholar
Gscholar
Authors’ Info
Authors’ Affiliation
Yoshiyuki Kinose
Takeshi Izuta
Institute of Agriculture, Tokyo University of Agriculture and Technology, Fuchu, Tokyo 183-8509 (Japan)
Graduate Faculty of Interdisciplinary Research, University of Yamanashi, Kofu, Yamanashi 400-8510 (Japan)
Corresponding author
Paper Info
Citation
Watanabe M, Kinose Y, Izuta T (2018). Photosynthesis of three evergreen broad-leaved tree species, Castanopsis sieboldii, Quercus glauca, and Q. myrsinaefolia, under elevated ozone. iForest 11: 360-366. - doi: 10.3832/ifor2493-011
Academic Editor
Silvano Fares
Paper history
Received: May 17, 2017
Accepted: Feb 25, 2018
First online: May 04, 2018
Publication Date: Jun 30, 2018
Publication Time: 2.27 months
Copyright Information
© SISEF - The Italian Society of Silviculture and Forest Ecology 2018
Open Access
This article is distributed under the terms of the Creative Commons Attribution-Non Commercial 4.0 International (https://creativecommons.org/licenses/by-nc/4.0/), which permits unrestricted use, distribution, and reproduction in any medium, provided you give appropriate credit to the original author(s) and the source, provide a link to the Creative Commons license, and indicate if changes were made.
Web Metrics
Breakdown by View Type
Article Usage
Total Article Views: 46893
(from publication date up to now)
Breakdown by View Type
HTML Page Views: 40371
Abstract Page Views: 2697
PDF Downloads: 2955
Citation/Reference Downloads: 10
XML Downloads: 860
Web Metrics
Days since publication: 2615
Overall contacts: 46893
Avg. contacts per week: 125.53
Article Citations
Article citations are based on data periodically collected from the Clarivate Web of Science web site
(last update: Mar 2025)
Total number of cites (since 2018): 4
Average cites per year: 0.50
Publication Metrics
by Dimensions ©
Articles citing this article
List of the papers citing this article based on CrossRef Cited-by.
Related Contents
iForest Similar Articles
Research Articles
A comparison between stomatal ozone uptake and AOT40 of deciduous trees in Japan
vol. 4, pp. 128-135 (online: 01 June 2011)
Research Articles
Evergreen species response to Mediterranean climate stress factors
vol. 9, pp. 946-953 (online: 07 July 2016)
Research Articles
A new approach to ozone plant fumigation: The Web-O3-Fumigation. Isoprene response to a gradient of ozone stress in leaves of Quercus pubescens
vol. 1, pp. 22-26 (online: 28 February 2008)
Research Articles
Successional leaf traits of monsoon evergreen broad-leaved forest, Southwest China
vol. 10, pp. 391-396 (online: 16 March 2017)
Research Articles
The use of branch enclosures to assess direct and indirect effects of elevated CO2 on photosynthesis, respiration and isoprene emission of Populus alba leaves
vol. 1, pp. 49-54 (online: 28 February 2008)
Research Articles
Drought tolerance in cork oak is associated with low leaf stomatal and hydraulic conductances
vol. 11, pp. 728-733 (online: 06 November 2018)
Short Communications
Variation in growth, photosynthesis and water-soluble polysaccharide of Cyclocarya paliurus under different light regimes
vol. 10, pp. 468-474 (online: 04 April 2017)
Research Articles
Adjustment of photosynthetic carbon assimilation to higher growth irradiance in three-year-old seedlings of two Tunisian provenances of Cork Oak (Quercus suber L.)
vol. 10, pp. 618-624 (online: 17 May 2017)
Research Articles
Adaptability of Indocalamus decorus to climate change based on physiological and biochemical responses to elevated carbon dioxide and ozone
vol. 9, pp. 311-317 (online: 22 October 2015)
Research Articles
Light acclimation of leaf gas exchange in two Tunisian cork oak populations from contrasting environmental conditions
vol. 8, pp. 700-706 (online: 08 January 2015)
iForest Database Search
Search By Author
Search By Keyword
Google Scholar Search
Citing Articles
Search By Author
Search By Keywords
PubMed Search
Search By Author
Search By Keyword