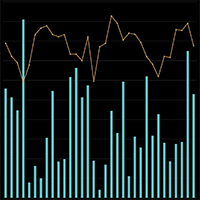
Net ecosystem production of a tropical secondary forest in Jengka, Pahang, Malaysia
iForest - Biogeosciences and Forestry, Volume 18, Issue 2, Pages 54-60 (2025)
doi: https://doi.org/10.3832/ifor4352-017
Published: Apr 04, 2025 - Copyright © 2025 SISEF
Research Articles
Abstract
Secondary tropical forests are important contributors to atmospheric carbon exchanges in tropical regions. However, our understanding of how secondary succession affects carbon cycling and carbon sequestration in these ecosystems is limited. We studied carbon cycling and net ecosystem production (NEP) in a secondary lowland forest using a full biometric-based flux measurement (2018-2019) and a partial biometric-based measurement (2017). Net primary production ranged from 6.01 to 8.37 Mg C ha-1 year-1 (2018-2019). This was partitioned in changes in aboveground biomass (0.98-3.66 Mg C ha-1 year-1), coarse roots (0.23-0.88 Mg C ha-1 year-1), litterfall (2.74-3.94 Mg C ha-1 year-1) and fine roots (0.86-1.09 Mg C ha-1 year-1). The total annual soil surface CO2 efflux was 10.56, 8.90, and 12.23 Mg C ha-1 year-1 for 2017, 2018, and 2019. The net ecosystem production of the forest was -2.89 and -3.86 Mg C ha-1 year-1 for the years 2018 and 2019. Litterfall exhibited the highest percentage of change (32%-58%) followed by aboveground biomass (14%-44%) and fine roots (13%-14%). The negative NEP values showed that the site is a C source and may be partially influenced by forest fragmentation. Seasonal variations also influence the dynamics of NPP and NEP.
Keywords
Carbon Cycling, Net Primary Production, Seasonal Variations, Tropical Secondary Forest, Soil Efflux
Introduction
Secondary forests are defined as forests essentially regenerating through natural processes after significant human and/or natural disturbance of the original forest vegetation at a single point in time or over an extended period. They can display a major difference in forest structure and/or canopy species composition with respect to nearby primary forests on similar sites ([9]). Carbon cycling in a forest varies during stand development, and the carbon sequestration rate is influenced by the forest’s age and successional stage ([40]). Woody biomass increments of stems and branches in secondary forests are essential components of carbon sequestration in forest ecosystems, and their functions as sinks/sources are gravely underestimated in the carbon cycle. Moreover, there is uncertainty about how carbon is partitioned and accumulates in forest ecosystems. Net Ecosystem Production and/or carbon accumulation (NEP) is a fundamental property of ecosystems. It was originally defined by Woodwell & Whittaker ([52]) as the difference between the amount of organic carbon fixed by photosynthesis (gross primary production, GPP) in an ecosystem, and total ecosystem respiration (ER). Ecosystem respiration encompasses the total respiration in an ecosystem, including both autotrophic respiration (by plants) and heterotrophic respiration (by decomposers and other organisms). Thus, NEP essentially represents the net amount of carbon that is either accumulated in or lost from it by export or non-biological oxidation (NEP = GPP-ER - [43]).
Net Primary Production (NPP) is the amount of organic carbon that remains after accounting for the carbon respired by plants (autotrophic respiration, AR). Thus, NPP = GPP - AR. This study used the biometric method to measure the yearly changes in NPP and carbon sequestration. Alternatively, the eddy covariance (EC) method is widely used for estimating CO2 fluxes and other gases between the atmosphere and forest ecosystems. This technique provides continuous data on the exchange of gases and is particularly useful for estimating GPP and ER over time. However, EC is known to be expensive, requires periodic visits to field sites and may underestimate nighttime respirations; also, ways to remove this bias remains controversial ([6], [4]). Compared with chronosequence studies of net CO exchange using the eddy covariance method ([44], [28]), there have been few studies on ecological inventories of carbon cycling and biometrically based flux measurements in the secondary forests ([54]).
Nevertheless, both methods of eddy covariance and biometric-based NEP provide process-level information on forest C-dynamics, in addition to validating localized data and regional estimates. Net ecosystem production of a forest can vary due to seasonal variations in gross primary production or unexpected defoliation ([20], [35], [45]), and this can concurrently impact the global carbon cycle. Despite the literature on inter-annual variations captured by long-term flux measurements is substantial ([53], [29]), data on biometric-based NEP needs to be explored, as both techniques may expose different factors that control C dynamics. To our knowledge, there has been no attempt to estimate NEP in Peninsular Malaysia, in contrast to the abundant literature that can be found for Indonesia ([5]), Vietnam ([51]), and China ([50]). Thus, understanding the variations in NEP on a larger spatial scale is still limited due to these circumstances. We hypothesize that measurements of NEP in a secondary forest will provide new insights into carbon balance and variables that need to be addressed for national or regional scale carbon modeling initiatives.
Our objectives were to estimate initial two-year measurements of biometric-based NEP in Jengka Forest Reserve and the contribution of different C fractions in a biological ecosystem that influences the C budget.
Materials and methods
Site description
The research was conducted in a secondary lowland forest in Pahang, West Malaysia. This site was chosen as data were available from a previous study ([23]). The experimental site is known as Jengka Virgin Jungle Reserve (Jengka VJR), Jengka 18, Pahang (03° 34.99′ N, 102° 34.29′ E) located at 50-90 m a.s.l. with slope ranging from 2-8 degrees on a flat terrain. Portions of the Jengka VJR were logged once between 1968 and 1969. It is a secondary forest with minimal disturbances, as selective loggings were done only in the 1960s ([31]). The soil type recorded here is silty clay loam from the Durian Series (Typic Paleudult). The complete description of soil analysis and results can be found in Jeyanny et al. ([21]). Six plots measuring 10 × 10 m were set up on site. The major botanical families are Phyllanthaceae, Euphorbiaceae, and Dipterocarpaceae, with trees ranging from 4 to 50 m in height (mean: 9.2 m) and dbh (diameter at breast height) ranging from 5 to 70 cm (mean: 10.2 cm). The common genera are Shorea, Aporosa, and Croton (Tab. 1). Our initial observations showed very little deadwood at the site, which may be a flux component. The wet season for the experimental site usually occurs from November to March, whereas the dry season occurs from May to September. Distinctive wet periods were recorded from October 2017 to January 2018, September 2018 to December 2018 and October-December 2019 (Fig. 1). In the period between October 2017 to June 2020, rainfall and temperature at Jengka VJR ranged from 20 to 455 mm and 26.0 to 28.5 °C (Fig. 1).
Tab. 1 - Dendromatic statistics of the study site at Jengka Forest Reserve (n = 82). Mean values ± standard errors are shown.
Variable | Values |
---|---|
Comon tree family | Phyllanthaceae, Dipterocarpaceae, Euphorbiaceae |
Common genera | Aporosa, Shorea, Croton |
Max dbh | 69.8 |
Mean dbh (cm) | 10.27 ± 1.13 |
Max height (m) | 50 |
Mean height estimated (m) | 9.2 ± 0.75 |
Stand basal area (m2 ha-1) | 22.8 ± 8.25 |
Trees (trees ha-1) | 1383 |
Fig. 1 - Monthly rainfall and air temperature at Jengka VJR forest, Malaysia from October 2017 to June 2020.
Experimental design and measurements for NEP
A transect running from North East towards South West was established in the study plot, whereby 60 quadrants measuring 10 × 10 m were established systematically, totaling 0.6 ha over the experimental site ([24]). Out of these 60 quadrants, six falling in the study plot were randomly selected to determine tree species using herbarium specimens and verified by a botanist. All stems with DBH (diameter at breast height) > 5 cm were measured and identified to species level in December 2017 using a diameter tape with the same staff during the research period to avoid human errors. The allometric equations used were described before in Jeyanny et al. ([22]). Living biomass was assessed in 2018 and 2019 following the same procedure, and the yearly increments in biomass (ΔM) were thus calculated.
Similarly, the coarse roots increment (ΔCr) was also estimated for 2018 and 2019. Coarse root increments were determined from the allometric equations developed for tropical ecological conditions by Niiyama et al. ([38]).
Twenty litter traps, 1 × 1 m in dimension and one meter above the ground, were systematically established within the plots to collect litterfall (Lf) every four months from 2018 to 2019. Litterfall samples were oven-dried at 80 °C, and the oven-dry mass was recorded periodically.
Measurement of fine roots (diameter ≤ 2 mm) involves root decomposition and fine root biomass (living and dead) to estimate fine root production. Root bags were prepared for the root decomposition study. To prepare root bags, fine roots were collected in bulk outside the designated plots amounting to 5 kg (fresh weight) of fine roots at 20 cm soil depth using a 150 mm in length and 80 mm in diameter soil coring probe. Roots were washed, air-dried, and oven-dried at 80 °C and inserted into 120 root bags of 1.0 g each. The root bags had a mesh opening size of 211 µm with a dimension of 10 × 10 cm (Sefar PET 1500), which blocked practically the ingrowth of fine roots; however, fine soil particles, water, and microorganisms can penetrate through the sheet. Root bags were buried at 10-15 cm soil depth and collected at corresponding time intervals every 4 months. Collected root bags were washed and then oven-dried for the remaining mass. The decomposition) was estimated as γij = (initial mass - remained mass)/initial mass. For each responding time interval, 18 root bags were collected up to 840 days (October 2017- February 2020). After collecting, root bags were air-dried and oven-dried at 80 °C for constant weight and recorded.
Sequence soil cores were collected every 4 months on the same dates with litter collection and other data to estimate fine root biomass. On each date, 30 soil cores were collected using the same stainless steel tube to a depth of 20 cm. The soil core positions were identified systematically in the main plot. To avoid errors, the soil corings points were positioned so as not to overlap with the root bag study, litterfall collections, and the soil respiration subplots. The collected soil was then washed and sieved using a 0.05 mm mesh size to collect fine roots. The living and dead roots were separated based on color, texture, and resilience ([19]). For example, dark/black colored roots that could break were deemed dead, and bright/yellow colored roots that were resilient were categorized as living roots. Afterward, the roots were air-dried ([19]) followed by oven-drying at 80 °C until a constant weight was obtained. Living roots and dead fine roots, together with the root decomposition ratio, were obtained to calculate the fine root production.
Soil surface respiration (Rs) is the production of carbon dioxide by organisms and plant parts in soil. The Rs is the sum of autotrophic respiration (Ra) of roots and rhizosphere organisms and heterotrophic respiration (Rh) of bacteria and fungi decomposition of organic matter and soil faunal activity in the organic and mineral horizons ([18]). Thus, to capture only Rh, trenched plots were prepared using a chopper in April 2017, 6 months before data collection. The dimensions were 1 × 1 m with a depth of 0.8 m. A six-month period before measurements was allowed for dead root decomposition to ensure that only Rh was recorded in trenched plots. Trenches were inspected periodically to remove the organic debris and clip intruding roots, so as to ensure that only heterotrophic respiration was recorded for the estimation of NEP.
Estimation methodology
Carbon accumulation of forest or net ecosystem production (NEP) in a forest ecosystem was simply estimated by using eqn. 1 ([39]), which assumes that inputs of C other than net primary production (NPP) and losses of C other than heterotrophic respiration (Rh) are negligible (eqn. 1):
NPP Estimation
NPP includes four components, namely, the aboveground living biomass increment (ΔM), the coarse root increment (ΔCr), the aboveground litterfall (Lf), and the fine root production (Lf) (eqn. 2):
Firstly, the aboveground biomass (AGB, kg dry weight) of each stem was estimated based on allometry according to Chave et al. ([8]), a well-known method for tropical forests receiving 1500-4000 mm annual rainfall, based on the diameter at breast height (DBH) and the wood-specific gravity (ρ). DBH was measured for the study period, while species average ρ was obtained from the Global Wood Density Database. The equation was fitted for each tree in the plots estimated. Finally, the value for each plot was totaled to obtain the aboveground biomass of carbon and extrapolated to one hectare to facilitate calculations as (eqn. 3):
where AGB is the aboveground biomass (kg) and d = ln(DBH).
The coarse roots were also estimated using the allometric equation by Niiyama et al. ([38]), which is suitable for Malaysian ecological conditions. Coarse roots were defined as ≥ 2 mm in diameter and estimated as (eqn. 4):
where WB is the belowground biomass and d is the diameter at breast height (1.3 m).
Estimation of aboveground and coarse roots increment
The change in the aboveground biomass (AGB, in kg) between 2017, 2018 and 2019 were estimated by calculating the variation ΔM, following Clark et al. ([10]) - eqn. 5):
where AGBa-tj and AGBa-tj are the AGB of stem a at time tj and ti, respectively, iAGBb-tj is the ingrowth of stem b at time tj , dAGBb-ti is the ingrowth of stem b at dbh = 5 cm, and tj > ti. The variation in coarse root increment (ΔCr) for the given years were estimated in a similar way (eqn. 6):
Aboveground litterfall
Lf (including all falling materials as leaves, branches, productive organs etc.) was estimated using 20 litter traps which were collected and totalled over the years 2018 and 2019.
Fine root production and turnover
Pr (fine root production) was estimated through the dry mass of living roots and dead roots at all collected dates ([42] - eqn. 7):
where Bi and Bj are the masses of living fine roots ≤ 2 mm in diameter (biomass) at time ti and tj, respectively (tj > ti), Ni and Nj are the masses of dead fine roots (necromass), and γij is the decomposition ratio of dead fine-roots at a corresponding time interval. Bi, Bj, Ni, and Nj were obtained by soil core sampling and γij was calculated by using the root bag technique (see above). Fine root turnover (FrT) was calculated according to Kubisch et al. ([30]) - eqn. 8):
where FrP is the annual belowground fine root production and sFrB is the standing belowground fine root biomass.
Soil respiration measurement
An automated soil CO2 flux system (LI-8100, Li-COR Biosciences, Lincoln, NC, USA) was used to measure soil CO2 fluxes to the atmosphere. Measurements were taken between 10:00 and 12:00 pm when daily soil CO2 fluxes were assumed to be at the highest rate ([34]). Periodic nighttime soil respiration was not accounted for, as random measurements done in October 2018 and February 2018 showed minimal diurnal variations. All measurements were done in 8 trenched plots, accounting for soil heterotrophic respiration only and not root respiration. Before measurements, soil collars with a 10 × 10 cm dimension were placed on the soil surface and inserted into the soil, leaving a headspace of 3 cm. Soil disturbance during collar insertion was minimized. During measurement, the chamber lid was closed, and the air was circulated in a gap between the collar headspace and the chamber. Once the CO2 concentration in the chamber had stabilized (approximately 30 seconds), the concentration was recorded for about 60 seconds. After every measurement, the automated soil CO2 flux system was moved from one sampling point to another. Each measurement was repeated twice at each sampling point. The systems software determined the flux rate by calculating the initial slope of a fitted exponential curve at the ambient CO2 concentration and given in units of µg mol CO2 m-2 s-1. These values were converted to mg CO2 m-2 h-1 by mathematical functions. Ancillary variable such as water-filled pore space (WFPS) was calculated from the soil water content and bulk density ([24]) and ranged between 52.80% and 57.97%
Conversion to Carbon
NPP was first estimated as dry biomass (Mg ha-1 year-1), then converted to carbon (Mg C ha-1 year-1), which equals 50% of dry biomass ([47]). This was done for ΔM, ΔCr, and Lf and Pr. Meanwhile, Rh was measured as µg mol CO2 m-2 s-1, then converted to carbon (Mg C ha-1 year-1). The difference between NPP and Rh is the carbon accumulation (Mg C ha-1 year-1), from which NEP was finally derived.
Statistical analysis
The growth variables of the sampled trees according to tree families were computed using descriptive statistics. Spatial variations of M and Cr were also quantified. All data corresponding to the time intervals were estimated and calculated separately according to individual trees and reported as means with standard errors for the years 2017 to 2019. However, mean values of Lf and Pr were computed similarly in separate plots and reported for the years 2018 and 2019 only. The total NPP was computed by summing up ΔM, ΔCr, ΔLf, and ΔPr for the years 2018 and 2019 respectively. The sum of NPP, according to the years determined, was subtracted from the annual heterotrophic respiration (Rh), and the NEP for 2019 and 2018 was reported.
Results and discussion
The primary botanical families (Tab. 1) recorded of trees were Phyllanthaceae, Euphorbiaceae, and Dipterocarpaceae. Trees ranged from 4 to 50 m in height (median: 8 m), and DBH ranged from 5 to 70 cm (median: 8.35 cm). The stand basal area was 22.8 m2 ha-1. Common genera were Shorea, Aporosa and Croton.
We conducted a complete biometric-based flux measurement over 2 years (2018-2019) and a partial biometric-based measurement (2017) in the Jengka Forest Reserve. Based on our results, it was displayed that there was a slight increment in M for the years 2018 to 2019 compared to 2017, whereby the highest was between 2018 and 2019, close to 3.66 Mg C ha-1 year -1 (Tab. 2). The Cr fraction was very minimal, less than 1 Mg C ha-1 year -1 and the Lf fraction showed values between 2.74 to 3.94 Mg C ha-1 year -1 for 2 years.
Tab. 2 - Mean ± standard error of Net Primary Production (NPP) and Net Ecosystem Production (NEP) at the Jengka Forest Reserve, Malaysia.
Variables | Mg C ha-1 year-1 | NPP changes | Ratio (%) |
|||
---|---|---|---|---|---|---|
2019 | 2018 | 2017 | 2018 | 2019 | ||
Aboveground biomass (M) | 96.53 ± 27.53 | 92.87 ± 27.71 | 91.89 ± 27.78 | 0.98 | 3.66 | 14-44 |
Coarse Roots (Cr) | 34.07 ± 12.96 | 33.19 ± 13.04 | 32.96 ± 13.07 | 0.23 | 0.88 | 3-10 |
Litterfall (Lf) | 2.74 ± 0.01 | 3.94 ± 0.02 | nd | 3.94 | 2.74 | 32-58 |
Fine roots production (Pr) | 1.09 | 0.86 | nd | 0.86 | 1.09 | 13-14 |
Total | - | - | - | 6.01 | 8.37 | - |
Soil heterotrophic respiration (Rh) | 12.23 ± 0.56 | 8.9 ± 0.56 | 10.56 ± 0.24 | - | - | - |
Net ecosystem Production (NEP) | -3.86 | -2.89 | nd | - | - | - |
The total NPP was 6.01-8.37 Mg C ha-1 year -1 (Tab. 2), whereby Lf accounted for the highest percentage (32%-58%) followed by M (14%-44%), and Pr (13%-14%). Based on the results, the abundant litterfall dominated the C budget, contributing to the soil organic matter pool that further allowed suitable woody increment in aboveground biomass via C mineralization/uptake. Van Do et al. ([51]) reported similar trends where Lf and Fr constituted the biggest portions of evergreen broad leave forests in Japan.
Fine root production (Pr) slightly increased (0.23 Mg C ha-1 year -1). Our results for coarse roots are higher than those reported by Ngo et al. ([37]) (18.8 Mg C ha-1) but consistent with those reported by Lü et al. ([33]) for aboveground biomass (136 Mg C ha-1) and coarse roots (38.6 Mg C ha-1) in a tropical forest ecosystem in Xishuangbanna, Southwestern China.
Before converting to C fractions, the fine root biomass production (FrP) for 2018 and 2019 were 627.80 and 791.05 g m-2 yr-1, respectively (data not shown). Still, upon conversion to tonnes ha-1, it constitutes 1.72 ± 0.03 t ha-1 yr-1 (2018) and 2.17 ± 0.07 t ha-1 yr-1 (2019), as shown in Fig. 2. Generally, the fine roots mortality (FrM) were 2.34 ± 0.06 t ha-1 yr-1 (2018) and 1.93 ± 0.05 t ha-1 yr-1 (2019). The fine root decomposition fraction (Frd) was 1.60 ± 0.002 t ha-1 yr-1 (2018) and 1.40 ± 0.003 t ha-1 yr-1 (2019) for the same period. The fine root turnover (FrT) calculated for 2018 and 2019 was 0.71 t yr-1. These values are similar to those reported by Gill & Jackson ([16]) for tropics, ranging from 0.60 to 0.75 t yr-1 in broadleaf tropical forests of Meghalaya, India ([3]) and Acacia saligna forest, ranging from 0.87 to 1.97 t yr-1, in Northern Kenya (Lehmann & Zech ([32]). The mean C allocation for fine roots in 2018 and 2019 was 0.86 and 1.09 yr-1 Mg C ha-1, respectively (Tab. 2).
Fig. 2 - Fine roots production (FrP), mortality (FrM), decomposition (Frd) and turnover (FrT) in 2018 and 2019. Error bars indicate standard errors (n=3).
In 2019 FrP was higher than FrM and Frd, and this was due to enhanced root decomposition (Frd), which increased root mortality in 2018, but it adversely affected FrP rates. The situation was reversed in the following year (Fig. 2). However, values for FrT were unchanged for both years. Approximately 70% of annual roots turned, consistent with those reported by Jiménez et al. ([25]) and Silver et al. ([49]) for tropical forests. When FrT is higher, it depicts its essential role in increased nutrient transformation rates for plant uptake, its control in fine root senescence dynamics, and the steady-state conditions of the study site ([16]). Our results for Pr were approximately half the Pr reported by Kho et al. ([27]) in Lambir, Sarawak, Malaysia (1.96 Mg C ha-1 yr-1). Our results for FrP were also higher (621.80-791.05 g m-2 yr-1) as compared to a meta-data analysis done for tropical forest (372.00 g m-2 yr-1) by Finér et al. ([13]) for sequential coring. The different values in 2018 and 2019 implied inter-annual variability of C fluxes ([49]) and the possibility of variable lifespans of fine roots ([15]) at this study site.
In this study, the mean annual estimated Rs were highest in 2019 (12.23 ± 0.56 Mg C ha-1 yr-1), followed by 2017 (10.56 ± 0.24 Mg C ha-1 yr-1) and 2018 (8.90 ± 0.56 Mg C ha-1 yr-1). The complete information on the partitioning of heterotrophic and autotrophic respiration can be found in Jeyanny et al. ([24]). Soil heterotrophic respiration (Rh) ranged between 518.60-784.08 mg CO2 m-2 h-1 ([24]), which upon conversion is between 8.90 and 12.23 Mg C ha-1 yr-1.
Our results showed a negative NEP, which indicates that Jengka FR was a source of carbon emission between 2018 and 2019 (Tab. 2), despite the high contributions from litterfall (Lf) and aboveground biomass (M) controlling the NPP. The results were also driven by high heterotrophic respiration. This was also possibly due to the specific characteristics of the location, which has been transformed into a fragmented forest with time, surrounded in adjacent areas by other crop plantations such as rubber and palm oil. Any drastic changes in these adjacent areas, such as the removal of vegetation, the introduction of water-demanding crops, and land use change, may affect the fragmented portion of forest in terms of environmental variables such as local air, soil temperatures and soil moisture retention in the forest site ([12]), thus relatively impacting the above ground biomass C density. Some trees at the forest edges experienced mortality due to wind throws. Logically, with increased soil temperatures and organic matter availability (i.e., from fallen trees and litter debris), the decomposition of fine roots and organic matter is expected, reducing the soil C stock in these areas. Emerging studies have shown that forest edges and fragments may only retain half as much of C as the forest interior ([36]), and this may have been one reason for the negative NEP obtained. Our study did not differentiate between edges and interior forest, and this is an emerging factor that has to be addressed in the future when reporting the C dynamics of a forest.
New studies reported that edge effects can translate into annual carbon emissions of 450-550 million Mt C year-1 ([14]). It was estimated that total carbon losses due to fragmented forests in the tropics globally is about 0.34 Gt C year-1 ([7]). Similar results were also reported for negative NEP (Tab. 3), such as in Pasoh by Adachi et al. ([2]) and Yoneda et al. ([54]) (-1.9 and -5.0 to 2.1 Mg C ha-1 year-1, respectively) and in Kalimantan, Indonesia by [5] (-0.1 Mg C ha-1 year-1). The typical results obtained were between -5.0 to 6.6 Mg C ha-1 year-1 based on the literature (Tab. 3). Scientists do agree that very poor data are available on carbon budgets related to fragmented forests and further explorations are needed ([36]). Thus, it is important to note that carbon losses in the tropics, driven by external sources such as fragmented forests, may have experienced a change in microclimatic conditions, abundance of organic matter debris, shifts in species composition, and ecosystem functionality ([55]). Inter-annual temporal patterns ([26], [24]) also contributed to the changes in NPP and NEP. The results obtained for Pr, Lf, Cr, M, and Rh were similar to the findings for the Malaysian moist tropical forests such as in Pasoh ([1]), Sarawak ([26]), and Sabah ([46]).
Tab. 3 - Net Ecosystem Production and measuring periods of selected forests.
Forest type | Study location | NEP (Mg C ha -1 y -1 ) |
Measuring period |
Sources |
---|---|---|---|---|
Evergreen old- growth broad-leaved forest | 21° 23′ N, 103° 38′ E (Northwest Vietnam) |
2.6 | 2014-2015 | [11] |
Evergreen secondary broad-leaved forest | 6.6 | |||
Broad black spruce (Picea mariana) forest | 54° N, 105° W (Saskatchewan, Canada) |
-1.3 | - | [39] |
Cool-temperate deciduous broad-leaved forest | 36° 08′ N, 137° 25′ E (Central Japan) |
2.1 | 1999-2003 | [40] |
2.4 | 1994-2003 | [45] | ||
A young larch forest | 62°13′ N, 129° 10′ E (Yakutsk, Russia) |
2.4 | 1998-1999 | [48] |
Japanese red pine (Pinus densiflora) stand | 35° 27′ N, 138° 46′ E (Mountain Fuji, Japan) |
2.9 | 1999-2008 | [41] |
Jack pine and aspen stands | 55° 51′ N, 98° 29′ E (Manitoba, Canada) |
1.0 | 2003 | [17] |
Dense stand of 500 cm tall jack pine | 1.7 | 2003 | ||
Primary rainforest | 2° 5′ N, 102° 18′ E (Pasoh Forest Reserve, Malaysia) |
-1.9 | 2004 | [2] |
Primary rainforest | 2° 5′ N, 102° 18′ E (Pasoh Forest Reserve, Malaysia) |
-5.0 to 2.1 | 1969-2012 | [54] |
Secondary rainforest | 3° 35′ N, 102° 34′ E (Jengka Virgin Jungle Reserve) |
-3.4 | 2017-2019 | This study |
Dry evergreen forest | 14° 30′ N, 101° 55′ E (Sakaerat Environmental Research Station,Thailand) |
0.7 | 2004 | [2] |
Previously logged peatswamp forest | Ketapang, West Kalimantan, Indonesia | -0.1 | 2014-2015 | [5] |
Conclusions
In this study, the NEP values estimated for a tropical secondary forest in Malaysia were negative, indicating that the study area was a source rather than a sink of carbon due to direct and indirect drivers related to inter-annual variability and forest fragmentation. The most significant fraction that contributed to NPP changes were the above-ground biomass and the litterfall. The dynamics of fine root production changed according to seasonal variability; in this forest, the production was lower compared to mortality and decomposition. We strongly believe that future work in C dynamics should explore differences in an intact forest, forest edges, and modified forest landscape with a long-term monitoring approach for refined estimates of NEP. This work may be a foundation for conservation strategies related to REDD+ initiatives and offer important information for the development and validation of C density models in fragmented forests on a national and regional scale.
Acknowledgments
We are indebted to the Forestry Department of Pahang for approving the field site study. This study was financially supported by the International Foundation of Science-South East Asian Regional Center for Graduate Study and Research in Agriculture (IFS-SEARCA) Collaborative Research Fund (J-1-D-6024-1). We sincerely appreciate the 11th Malaysia Plan funding to Forest Research Institute Malaysia to facilitate the acquisition of the funding for Li-8100A automated soil flux system data collection at the study site. Thanks are due to the Forest Research Institute Malaysia staff for field and technical assistance.
References
CrossRef | Gscholar
Gscholar
Gscholar
CrossRef | Gscholar
CrossRef | Gscholar
CrossRef | Gscholar
Gscholar
CrossRef | Gscholar
Authors’ Info
Authors’ Affiliation
Muhammad Asri Lias 0000-0002-6383-9247
Mohd Syamin Aizat Mohd Yusof 0009-0006-1390-0739
Mohd Zaki Abdullah 0000-0002-3191-3780
Tran Van Do 0000-0001-9059-5842
Wan Rasidah Kadir
Forest Research Institute Malaysia (FRIM), 52109 Kepong, Selangor Darul Ehsan (Malaysia)
Corresponding author
Paper Info
Citation
Vijayanathan J, Lias MA, Mohd Yusof MSA, Abdullah MZ, Van Do T, Kadir WR (2025). Net ecosystem production of a tropical secondary forest in Jengka, Pahang, Malaysia. iForest 18: 54-60. - doi: 10.3832/ifor4352-017
Academic Editor
Giorgio Alberti
Paper history
Received: Mar 22, 2023
Accepted: Nov 21, 2024
First online: Apr 04, 2025
Publication Date: Apr 30, 2025
Publication Time: 4.47 months
Copyright Information
© SISEF - The Italian Society of Silviculture and Forest Ecology 2025
Open Access
This article is distributed under the terms of the Creative Commons Attribution-Non Commercial 4.0 International (https://creativecommons.org/licenses/by-nc/4.0/), which permits unrestricted use, distribution, and reproduction in any medium, provided you give appropriate credit to the original author(s) and the source, provide a link to the Creative Commons license, and indicate if changes were made.
Web Metrics
Breakdown by View Type
Article Usage
Total Article Views: 2788
(from publication date up to now)
Breakdown by View Type
HTML Page Views: 997
Abstract Page Views: 782
PDF Downloads: 849
Citation/Reference Downloads: 0
XML Downloads: 160
Web Metrics
Days since publication: 118
Overall contacts: 2788
Avg. contacts per week: 165.39
Article Citations
Article citations are based on data periodically collected from the Clarivate Web of Science web site
(last update: Mar 2025)
(No citations were found up to date. Please come back later)
Publication Metrics
by Dimensions ©
Articles citing this article
List of the papers citing this article based on CrossRef Cited-by.
Related Contents
iForest Similar Articles
Research Articles
Soil CO2 efflux in uneven-aged and even-aged Norway spruce stands in southern Finland
vol. 11, pp. 705-712 (online: 06 November 2018)
Research Articles
Effect of different dolomitic limestone dosages on soil respiration in a mid-altitudinal Norway spruce stand
vol. 12, pp. 357-365 (online: 05 July 2019)
Research Articles
Different harvest intensity and soil CO2 efflux in sessile oak coppice forests
vol. 9, pp. 546-552 (online: 25 March 2016)
Research Articles
Estimating changes in soil organic carbon storage due to land use changes using a modified calculation method
vol. 8, pp. 45-52 (online: 17 June 2014)
Research Articles
The manipulation of aboveground litter input affects soil CO2 efflux in a subtropical liquidambar forest in China
vol. 12, pp. 181-186 (online: 10 April 2019)
Research Articles
Temporal patterns control carbon balance in forest and agricultural tropical peatlands in North Selangor, Malaysia
vol. 14, pp. 362-369 (online: 10 August 2021)
Research Articles
Soil respiration along an altitudinal gradient in a subalpine secondary forest in China
vol. 8, pp. 526-532 (online: 01 December 2014)
Review Papers
Recent insights in soil nutrient cycling: perspectives from Pinus and Eucalyptus forest studies around the world
vol. 17, pp. 394-404 (online: 20 December 2024)
Research Articles
Soil respiration and carbon balance in a Moso bamboo (Phyllostachys heterocycla (Carr.) Mitford cv. Pubescens) forest in subtropical China
vol. 8, pp. 606-614 (online: 02 February 2015)
Research Articles
Contribution of legume and non-legume trees to litter dynamics and C-N-P inputs in a secondary seasonally dry tropical forest
vol. 15, pp. 8-15 (online: 13 January 2022)
iForest Database Search
Search By Author
Search By Keyword
Google Scholar Search
Citing Articles
Search By Author
Search By Keywords
PubMed Search
Search By Author
Search By Keyword