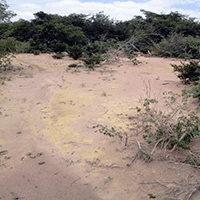
Impact of deforestation on the soil physical and chemical attributes, and humic fraction of organic matter in dry environments in Brazil
iForest - Biogeosciences and Forestry, Volume 15, Issue 6, Pages 465-475 (2022)
doi: https://doi.org/10.3832/ifor4016-015
Published: Nov 18, 2022 - Copyright © 2022 SISEF
Research Articles
Abstract
Deforestation of Caatinga and inadequate land use of these dry environments have impacted soil quality in Northeastern Brazil. The objectives of this study were: (a) to evaluate the effect of deforestation and different agricultural uses on the physical and chemical properties of soil, and humic fractions of soil organic matter in dry environments; and (b) to detect the soil properties that were most affected by anthropic actions. We evaluated four dry areas in Chapada do Araripe, NE Brazil: preserved native vegetation; degraded native vegetation; cassava conventional cultivation; and eucalyptus agro-energy cultivation. Soil fertility, total organic carbon and humic fractions of soil organic matter were lower in the degraded native vegetation area. The best indicators for soil quality evaluation were: macroporosity; bulk density; soil resistance penetration; sum of bases (mainly Ca2+); available P; and saturation by Al3+. Total organic carbon and humic acid fractions of soil organic matter were important in improving soil quality. These properties were influenced by deforestation and agricultural uses, suggesting that the deforestation of native vegetation in dry environments has high capacity to degrade the soil, preventing its regeneration.
Keywords
Introduction
Deforestation and inadequate use of soil in semi-arid regions, along with the unfavorable climatic conditions of these regions, poorly developed soils and low biomass production, influence the regenerative capacity of the vegetation and soil degradation. Therefore, the use of more efficient and sustainable management systems is necessary for mitigate these negative effects on vegetation and soil in these regions ([39], [40]). Mekuria et al. ([26]) highlighted the importance of studies evaluating soil degradation in semi-arid regions, though research on this subject is still incipient.
The gypsum mining in the Araripe region of the Brazilian semi-arid region presents a high biomass demand for the calciners, which is responsible for the high rates of deforestation, making this region susceptible to desertification and reducing the productive capacity of soils ([14]). According to Araújo Filho et al. ([3]), soil degradation in the Brazilian semi-arid region has been influenced by the removal of vegetation cover. Studies by Vieira et al. ([48]) indicated that the semi-arid region of the Northeast of Brazil is subject to a high degree of desertification, and the inadequate removal of vegetation cover is one of its main causes.
Changes in the environmental landscape and land use in the semi-arid region are also associated with agricultural expansion. Several studies showed that the agricultural uses in this region have caused soil degradation ([5], [39], [24]). Cassava is the main subsistence crop in Chapada do Araripe (Pernambuco State, NE Brazil) due to its widespread use in family agriculture. This agricultural use of the soil, together with the harvest of the native vegetation as a source of energy for the calcination of gypsum from mining have been the main causes of deforestation of the Caatinga.
The impact on forest resources in the Araripe region has led to the use of strategic alternatives to contain deforestation ([32]). Afforestation with eucalyptus plantations for biomass production to replace the native Caatinga vegetation has been one of these alternatives. Despite these environmental benefits, there is still little scientific information on the impact of the use of this exotic species on the quality of the soil in the region. Indeed, assessing these impacts and establishing an adequate management plan for land uses in this region can directly contribute to soil recovery and conservation, and can indirectly improve the quality of life of local people relying on these natural resources. Monitoring the impact of different land uses and assessing soil quality are keys for early diagnosis of environmental disturbances ([7]).
In this study, we hypothesized that the deforestation of Caatinga for cultivation of cassava monoculture or eucalyptus plantations for use as biomass energy may be the cause of environmental degradation in different soil properties responsible for maintaining the quality and sustainability of the environment.
Recently, soil degradation in dry environment has expanded in the Chapada do Araripe region, mainly due to the intense exploitation of native vegetation, thus making it essential to study the physical and chemical aspects of soil quality. In this study, we meant to answer the following questions: (i) which soil properties are being most impacted by deforestation? (ii) What is the influence of subsistence family farming (cassava cultivation) on soil properties? (iii) Can the establishment of alternative energy crops, such as eucalyptus, affect soil properties and reduce its quality?
Material and methods
Study area
The study was carried out in top areas of Chapada do Araripe (07° 27′ 32″ S and 40° 24′ 55″ W), located in the extreme west of the state of Pernambuco, in the municipality of Araripina, in an area of gypsum mining and production. According to the classification of Koppen ([2]), the climate of the region is warm semi-arid, steppe-type with summer-autumn rainfall. Mean annual precipitation is 77 mm and mean annual temperature over the last 30 years is 24 °C. The topography is flat, with altitude varying from 828 to 837 m a.s.l. The soil of the study areas was classified as Dystrophic Yellow Latosol ([31]), corresponding to Oxisol ([38]).
The native vegetation of the top areas of Chapada do Araripe includes the following dominant species: Guapira opposita (Vell.) Reitz; Croton limae A.P.S. Gomes, M.F. Sales & P.E. Berry; Metrodorea mollis Taub.; Pilocarpus spicatus subsp. aracatensis Kaastra; Annona leptopetala (R.E.Fr.) H. Rainer; Senegalia langsdorffii (Benth.) Seigler & Ebinger; Swartzia psilonema Harms; Zanthoxylum hamadryadicum Pirani; Byrsonima gardneriana A. Juss.; Piptademia viridiflora (kunth) Benth. ([30]).
The study was carried out in four distinct though adjacent areas: (i) preserved native vegetation area (PNV); (ii) degraded native vegetation area, with low natural regeneration (DNV); (iii) cassava conventional cultivation area (CCC), with plantations established 46 years ago; and (iv) eucalyptus agro-energetic cultivation area (EAC), aged only 11 years (Fig. 1). Information on land use history and management used in each study area was obtained through interviews to old farmers and local research institutions (Tab. S1 in Supplementary material).
Fig. 1 - The studied sites. (a) Preserved native vegetation (PNV); (b) degraded native vegetation (DNV); (c) Cassava conventional cultivation (CCC); (d) Eucalyptus agro-energy cultivation (EAC).
Data collection
The number of samples needed to accurately represent each soil property (sample adequacy) was calculated as follows ([37] - eqn. 1):
where N is the number of samples; tα is the t value from Student distribution for a probability α (p<0.05) and N - 1 degrees of freedom; f is the angular error in relation to the mean; and CV (=100 · standard deviation / mean) is the coefficient of variation of the soil property.
Physical properties
Sampling for determination of the physical properties was performed at the four vertices and in the center of a 100 × 100 m square plot located in the center of each study area, at depths of 0-10 cm and 10-20 cm, totaling ten samples per area (40 samples in total). Samples were collected in volumetric rings with 5 × 5 cm (height × diameter) without structure deformation. The tα value for the physical properties was 2.7764 for 4 degrees of freedom (5 samples) and probability α (p<0.05). The CV of each physical property was calculated and f values of up to 50% were used, as physical properties are less variable than chemical properties and SOM fractions.
The properties analyzed were total porosity (TP), macroporosity (MaP), microporosity (MiP), field capacity moisture content (FC), wilting point moisture content (WP), soil resistance penetration (SRP) and bulk density (BD). Available water (AW) content was calculated by the difference between FC and WP. A preliminary analysis revealed small and negligible variation in soil texture and particle size distribution among the different areas (Tab. S2 in Supplementary material).
Samples were saturated for 24 h for determination of TP, MaP and MiP. After the saturation period, samples were submitted to the tension of 6 KPa using a tension table for MiP determination. MaP was obtained by the difference between TP and MiP. To determine BD, samples were placed in an oven at 105 °C until constant weight. To obtain the FC, samples were placed on the tension table at 10 KPa, and to obtain the WP, samples were placed in a Richards extractor at 1.500 KPa ([12]). Samples were weighted after equilibration at 80 KPa. Then, determination of SRP was performed using an electronic penetrometer with a needle to simulate the penetration of the root at a speed of 1 cm min-1. The base of the cone was 4 mm thick ([9]).
Chemical properties
Sampling for determination of chemical properties, TOC and SOM fractions was performed within the 100 × 100 m square plot in the center of each study area. Twenty-five samples were collected within each plot, spaced 25 × 25 m at depths of 0-5 cm, 5-10 cm and 10-20 cm, totaling 75 samples per area (300 samples in total). Samples were collected with a Dutch type auger with a deformed structure. The tα value for these properties was 2.0639 for 24 degrees of freedom (25 samples) and probability α (p<0.05). The CV of each property was calculated and f values of up to 30% were considered ([18]).
The collection of undisturbed samples to evaluate the physical properties of the soil was carried out on the side of a small soil profile by introducing a volumetric ring of 5 cm in diameter. In this case, soil sampling was carried out at depths 0-10 cm (topsoil) and 10-20 cm (subsoil), as there was not enough space to collect samples at the same depths used for the evaluation of soil chemical properties (0-5 cm; 5-10 cm; and 10-20 cm - see above). Additionally, we sampled the entire A horizon and a fraction of the AB horizon, as the most effective absorption capacity of the root system is up to 20 cm deep.
The following chemical properties were determined on soil samples: pH in H2O; exchangeable Ca2+; Mg2+; K+; Al3+; potential acidity (H+Al); and available P. The pH was measured with a glass electrode in a 1:2.5 ratio of the soil solution in distilled water. The Ca2+, Mg2+ and Al3+ were extracted with KCl 1.0 mol L-1. Ions Ca2+ and Mg2+ were measured by atomic absorption spectroscopy and Al3+ was measured by titration in the presence of the blue bromothymol indicator and titrated with NaOH (0.025 mol L-1). Available P and K+ were estimation with Mehlich-1 extraction. P concentration was dosed by colorimetry and K+ content was dosed by flame photometry. The (H+Al) was extracted with 0.5 mol L-1 calcium acetate and titrated with NaOH and phenolphthalein as indicator. All chemical protocols were taken from Teixeira et al. ([46]).
The following indexes derived from the above chemical properties were obtained: sum of bases (SB); potential cation exchange capacity (CECp); saturation by bases (V) and saturation by aluminum (Al saturation).
Humic substances fractions of organic matter
TOC was determined by oxidation of the organic matter by potassium dichromate (K2Cr2O7) 0.020 mol L-1 and titrated with ammonium ferrous sulphate (Mohr’s salt) 0.005 mol L-1, according to Yeomans & Bremner ([50]).
Chemical fractionation of SOM was based on different solubilities of fulvic acid (FA-C), humic acid (HA-C) and humin (HU-C) fractions in acid and alkaline media, according to Swift ([42]). For this, 2.0 g of soil was immersed in 20 mL of 0.1 mol L-1 NaOH for 24 h. Separation between alkaline extract (FA-C + HA-C) and residue was performed by centrifugation at 3000×g for 30 min. The residue was washed again with 20 mL of the NaOH solution and centrifugation again at 3000×g for 30 min. This washing solution was mixed with the extract. The pH of the alkaline extract was adjusted to 1.0 (±0.1) using 20% H2SO4 before decantation for 18 h. The precipitate (HA-C) was separated from the soluble fraction (FA-C) by filtration. The material remaining in the centrifuge tubes was considered as the HU-C fraction. The fractions FA-C and HA-C was determined in 5 mL extract aliquots mixed with 1.0 mL of 0.042 mol L-1 potassium dichromate and 5 mL of concentrated H2SO4 in a block digester at 150 °C (30 min), followed by titration with 0.0125 mol L-1 ferrous ammonium sulfate. In the oven-dried residue, HU-C was determined by adding 5 mL of 0.1667 mol L-1 potassium dichromate and 10 mL of concentrated H2SO4 to a block digester at 150 °C (30 min) and titrating with 0.25 mol L-1 ferrous ammonium sulfate and using ferroin indicator solution. After determinations, the following humification indexes were derived: humic substances fractions (HS-C), ratio between HA-C/FA-C and ratio between HS-C/TOC. HS-C is the sum of HA-C, FA-C and HU-C.
Statistical procedures
Data from physical and chemical properties, TOC and SOM fractions were submitted to analysis of variance by the F test (p<0.05). When there was a significant effect of the different land uses on these properties, the Scott-Knott’s mean test (p<0.05) was applied.
Data were also analyzed by multivariate statistics, through principal component analysis (PCA) and cluster analysis (CA) to select properties that were most influenced by different land uses and to identify similar groups ([35]). The Ward’s algorithm was used as the agglomeration method and the Euclidean distance as measure of dissimilarity ([35]).
Data were centralized and normalized to mean zero and variance one to ensure that all properties equally contributed to the multivariate models used ([35]). In order to select the properties with greater variability, correlations with values of r ≥ 0.70 were considered as significant ([4]). This criterion considers that the number of principal components needed for the interpretation of the results is based on the explanation of at least 70% of the total variation.
Results
Soil physical properties
Different land uses had an effect on MaP, TP and BD in the soil surface layer (topsoil: 0-10 cm) and on SRP in both soil layers. The soil physical properties FC, WP AW were only influenced by different land uses in the subsurface layer (subsoil: 10-20 cm - Tab. 1).
Tab. 1 - Soil physical attributes of topsoil (0-10 cm) and subsoil (10-20 cm) under forest, deforested, cropland and agro-energy sites. (PNV): preserved native vegetation; (DNV): degraded native vegetation; (CCC): Cassava conventional cultivation; (EAC): Eucalyptus agro-energy cultivation; (MaP): macroporosity; (MiP): microporosity; (TP): total porosity; (BD): bulk density; (SRP): soil resistance penetration; (FC): field capacity moisture content; (WP): wilting point moisture content; (AW): available water. Mean values and standard deviation are displayed. Values in columns with similar letters are not significantly different (p>0.05); (ns): not significant.
Soil Depth |
Site | MaP (m-3 m-3) |
MiP (m-3 m-3) |
TP (m-3 m-3) |
BD (Mg m-3) |
SRP (Mpa) |
FC (m-3 m-3) |
WP (m-3 m-3) |
AW (m-3 m-3) |
---|---|---|---|---|---|---|---|---|---|
0-10 cm | PNV | 0.17 ± 0.03 a | 0.31 ± 0.01 a | 0.48 ± 0.02 a | 1.27 ± 0.06 b | 1.12 ± 0.22 b | 0.30 ± 0.01 a | 0.09 ± 0.01 a | 0.21 ± 0.02 a |
DNV | 0.08 ± 0.01 b | 0.33 ± 0.01 a | 0.41 ± 0.02 b | 1.51 ± 0.07 a | 3.44 ± 0.69 a | 0.33 ± 0.01 a | 0.10 ± 0.01 a | 0.22 ± 0.02 a | |
CCC | 0.13 ± 0.02 a | 0.36 ± 0.02 a | 0.49 ± 0.02 a | 1.34 ± 0.06 b | 0.86 ± 0.17 b | 0.36 ± 0.02 a | 0.10 ± 0.01 a | 0.25 ± 0.02 a | |
EAC | 0.16 ± 0.03 a | 0.33 ± 0.01 a | 0.49 ± 0.02 a | 1.30 ± 0.06 b | 1.07 ± 0.21 b | 0.32 ± 0.01 a | 0.10 ± 0.01 a | 0.22 ± 0.02 a | |
Mean | 0.15 ± 0.02 | 0.33 ± 0.01 | 0.46 ± 0.02 | 1.35 ± 0.07 | 1.62 ± 0.32 | 0.32 ± 0.01 | 0.09 ± 0.01 | 0.22 ± 0.02 | |
F-test | 4.05* | 3.37 ns | 4.28* | 3.78* | 26.16* | 3.20 ns | 1.00 ns | 1.33 ns | |
10-20 cm | PNV | 0.12 ± 0.02 a | 0.33 ± 0.01 a | 0.45 ± 0.01 a | 1.34 ± 0.02 a | 0.93 ± 0.14 b | 0.33 ± 0.01 b | 0.07 ± 0.003 b | 0.25 ± 0.01 a |
DNV | 0.08 ± 0.01 a | 0.35 ± 0.01 a | 0.43 ± 0.01 a | 1.43 ± 0.02 a | 2.05 ± 0.30 a | 0.35 ± 0.01 a | 0.10 ± 0.004 a | 0.25 ± 0.01 a | |
CCC | 0.10 ± 0.02 a | 0.36 ± 0.01 a | 0.47 ± 0.01 a | 1.41 ± 0.02 a | 1.46 ± 0.22 b | 0.36 ± 0.01 a | 0.10 ± 0.004 a | 0.27 ± 0.02 a | |
EAC | 0.10 ± 0.02 a | 0.33 ± 0.01 a | 0.42 ± 0.01 a | 1.42 ± 0.02 a | 2.69 ± 0.40 a | 0.32 ± 0.01 b | 0.10 ± 0.004 a | 0.21 ± 0.01 b | |
Mean | 0.10 ± 0.02 | 0.34 ± 0.01 | 0.44 ± 0.01 | 1.40 ± 0.02 | 1.78 ± 0.26 | 0.34 ± 0.01 | 0.09 ± 0.004 | 0.24 ± 0.01 | |
F-test | 1.60 ns | 14.28 ns | 2.14 ns | 3.49 ns | 10.52* | 16.03* | 16.19* | 17.73* |
The DNV area in the topsoil had the lowest values of MaP and TP and the highest values of BD and SRP. The DNV area presented the highest SRP value, being 207, 300 and 221% higher than the PNV, CCC and EAC areas. The PNV, CCC and EAC areas presented MaP values above 0.10 m3 m-3. The DNV area at both depths and EAC at the subsurface layer showed SRP values greater than 2.0 MPa (Tab. 1).
The physical properties that better accounted for data variation were MaP, TP, BD and SRP in topsoil, which were significantly correlated with PC1, showing the influence of the different soil uses on these properties. Regarding PC2, MiP, FC and AW showed the highest and significant correlation values with this axis (Tab. 2).
Tab. 2 - Correlation between principal components (PC1, PC2) and soil physical attributes of topsoil (0-10 cm) and subsoil (10-20 cm). (MaP): macroporosity; (MiP): microporosity; (TP): total porosity; (BD): bulk density; (SRP): soil resistance penetration; (FC): field capacity moisture content; (WP): wilting point moisture content; (AW): available water; (*): p<0.05.
Attribute | 0-10 cm | 10-20 cm | ||
---|---|---|---|---|
PC1 | PC2 | PC1 | PC2 | |
MaP | -0.983* | 0.027 | -0.647 | -0.568 |
MiP | 0.365 | 0.930* | -0.258 | 0.966* |
TP | -0.834* | 0.525 | -0.735* | 0.548 |
BD | 0.991* | -0.124 | 0.816* | 0.427 |
SRP | 0.851* | -0.520 | 0.997* | -0.074 |
FC | 0.487 | 0.864* | -0.252 | 0.959* |
WP | 0.692 | 0.530 | 0.935* | 0.276 |
AW | 0.234 | 0.955* | -0.695 | 0.713* |
Absolute variance (%) | 54.80 | 40.90 | 54.10 | 37.80 |
Cumulated variance (%) | 54.80 | 95.70 | 54.10 | 91.90 |
Regarding the 10-20 cm depth layer (subsoil), the first two PC axes explained 91.90% of the total variance in physical properties among the 4 different land uses. PC1 a 54.10% and presented a high correlation with TP, SRP, BD and WP, while PC2 accountd for 37.80% of the variability and showed a high correlation with MiP, FC and AW (Tab. 2).
In the derived PC biplots (Fig. 2), BD and SRP vectors were opposed to MaP and TP along the first PC axis in the top 0-10 cm layer (Fig. 2a). SRP was also inversely related to MiP, FC and AW in the 10-20 cm depth layer (Fig. 2b).
Fig. 2 - Biplots of the soil physical properties. (a) Topsoil (0-10 cm) and (b) subsoil (10-20 cm). (PNV): Preserved native vegetation; (DNV): Degraded native vegetation; (CCC): Cassava conventional cultivation; (EAC): Eucalyptus agro-energy cultivation. Soil physical attributes: (MaP) macroporosity; (MiP) microporosity; (TP) total porosity; (BD) bulk density; (SRP) soil resistance penetration; (FC) field capacity moisture content; (WP) wilting point moisture content; (AW) available water. The arrow represents the direction of high weighting (maximum variation) of soil physical properties along the principal components. PC1 and PC2 of topsoil (a) explain 95.7% of the variation, while PC1 and PC2 of subsoil (b) explain 91.9% of the total variation.
The different land uses sampled were distributed in different quadrants of the biplots in both layer of the soil, forming 4 distinct groups (Fig. 2). This shows that the different land uses have very different physical properties of the soil. For example, the DNV land use is characterized by extreme values of DB and SRP in the topsoil (0-10 cm - Fig. 2a) and by extreme values of BD and WP in the subsurface layer (10-20 cm - Fig. 2b). This means that soil bulk density (BD) is largely affected in the degraded native vegetation (DNV) as compared with the preserved native vegetation (PNV). Similarly, MaP and TP in the topsoil (0-10 cm) and SRP in the subsoil (10-20 cm) showed extreme values under the EAC land use (Fig. 2). Moreover, MiP, FC, WP and AW properties in the soil surface layer (Fig. 2a) had extreme values under the CCC land use, as well as TP, MiP, FC and AW in the subsurface layer (Fig. 2b). The preserved native vegetation (PNV) had the higher macroporosity values (MaP) in the subsurface layer (10-20 cm - Fig. 2b, Tab. 1), and in general it showed more balanced physical properties as compared with the other land use types.
Cluster analysis based on soil physical attributes highlighted the existence of three groups of land uses, according to the different types of land use and soil depth (Fig. 3). In the superficial layer, the first group was formed only by the DNV area, the second group was formed only by the CCC area and the third group joined the PNV and EAC areas (Fig. 3a). In the subsurface layer, DNV and CCC areas were grouped and PNV and EAC areas were separated and formed individual groups (Fig. 3b). The more intensive use of the soil in the CCC area showed degradation of its physical properties and evolution towards the levels of the degraded natural vegetation (DNV). The use of eucalyptus (EAC) was more conservative, preserving the favorable physical properties of the preserved area (PNV).
Fig. 3 - Dendrograms of the different land uses analyzed according to the soil physical properties. (a) Topsoil (0-10 cm) and (b) subsoil (10-20 cm).(PNV): preserved native vegetation; (DNV): degraded native vegetation; (CCC): Cassava conventional cultivation; (EAC): Eucalyptus agro-energy cultivation.
Soil chemical properties
Deforestation and different land uses altered soil chemical properties at all depths (Tab. 3). The pH values were generally higher in the CCC and EAC plots, except in the surface layer (0-5 cm depth), where the highest value was recorded in the EAC. The lowest pH values occurred in the DNV plot. The pH was also low in the PNV area, matching the DNV plot in the 10-20 cm depth layer (Tab. 3). The other components of soil acidity, such as Al3+ and Al saturation were higher in the DNV area at all depths (Tab. 3). The areas under agricultural cultivation had higher soil pH and lower Al saturation values, especially in superficial layers. However, the value of (H + Al) and CECp were higher in the PNV area (Tab. 3).
Tab. 3 - Soil chemical attributes of topsoil (0-5 cm) and subsoil (5-10 and 10-20 cm) under forest, deforested, cropland and agro-energy sites. (PNV): preserved native vegetation; (DNV): degraded native vegetation; (CCC): Cassava conventional cultivation; (EAC): Eucalyptus agro-energy cultivation. Soil chemical attibutes: (pH) hydrogen potential (H2O/1:2.5); (P) available phosphorus; (K+) exchangeable potassium; (Ca2+) exchangeable calcium; (Mg2+) exchangeable magnesium; (Al3+) exchangeable aluminum; (H+Al) potential acidity; (SB) sum of bases; (CECp) cations exchange capacity potential; (V) base saturation; (Al saturation) saturation by aluminum. Mean values and standard deviation are reported. Values in columns with similar letters are not significantly different (p>0.05).
Depth | Site | pH | P (mg dm-3) |
K+ (cmolc dm-3) |
Ca2+ (cmolc dm-3) |
Mg2+ (cmolc dm-3) |
Al3+ (cmolc dm-3) |
H+Al (cmolc dm-3) |
SB (cmolc dm-3) |
CECp (cmolc dm-3) |
V (%) |
Al saturation (%) |
---|---|---|---|---|---|---|---|---|---|---|---|---|
0-5 cm | PNV | 4.83±0.25b | 1.26±0.63b | 0.14±0.03a | 0.65±0.02b | 0.18±0.08b | .0.51±0.13b | 6.68±1.87a | 0.97±0.29c | 7.66±1.98a | 13.36±4.80b | 35.19±9.04b |
DNV | 4.49±0.20c | 0.95±0.68c | 0.06±0.01c | 0.36±0.05c | 0.07±0.02c | 0.66±0.12a | 4.15±1.05c | 0.52±0.08d | 4.67±1.04d | 11.70±3.25b | 55.70±6.03a | |
CCC | 4.96±0.38b | 2.10±0.63a | 0.10±0.03b | 0.98±0.19a | 0.18±0.06b | 0.38±0.09c | 4.27±1.14c | 1.29±0.25b | 5.57±1.14c | 24.17±6.67a | 23.23±7.23c | |
EAC | 5.42±0.51a | 2.59±0.89a | 0.14±0.07a | 1.04±0.38a | 0.32±0.18a | 0.33±0.17c | 4.78±0.79a | 1.53±0.57a | 6.31±0.79b | 24.19±8.51a | 19.94±13.43d | |
Mean | 4.92±0.34 | 1.72±0.71 | 0.11±0.03 | 0.75±0.16 | 0.19±0.09 | 0.47±0.13 | 4.97±1.21 | 1.07±0.30 | 6.05±1.24 | 18.35±5.81 | 33.51±8.93 | |
F-test | 25.97* | 25.47* | 16.24* | 40.91* | 22.94* | 37.72* | 24.56* | 36.49* | 25.32* | 30.75* | 79.04* | |
5-10 cm | PNV | 5.00±0.21b | 1.26±0.62b | 0.07±0.02b | 0.80±0.21b | 0.15±0.05b | 0.53±0.11b | 6.60±1.59a | 1.03±0.27b | 7.63±1.33a | 13.67±2.91b | 34.71±7.01b |
DNV | 4.72±0.12c | 0.81±0.66c | 0.03±0.01d | 0.45±0.06c | 0.04±0.01c | 0.60±0.10a | 4.48±0.94b | 0.53±0.07c | 5.02±0.97c | 10.93±1.99c | 52.78±5.02a | |
CCC | 5.23±0.32a | 2.04±0.69a | 0.05±0.01c | 1.04±0.21a | 0.18±0.08b | 0.36±0.11c | 4.98±1.29b | 1.29±0.24a | 6.27±1.33b | 21.16±4.85a | 22.37±8.01c | |
EAC | 5.22±0.46a | 1.78±0.91a | 0.09±0.05a | 1.00±0.37a | 0.28±0.11a | 0.36±0.16c | 5.16±1.00b | 1.39±0.56a | 6.55±1.05b | 21.37±7.76a | 22.82±12.90c | |
Mean | 5.04±0.28 | 1.47±0.72 | 0.06±0.02 | 0.82±0.21 | 0.16±0.06 | 0.46±0.12 | 5.30±1.21 | 1.06±0.29 | 6.37±1.17 | 16.78±4.38 | 33.17±8.24 | |
F-test | 15.70* | 15.13* | 14.89* | 28.36* | 20.95* | 27.70* | 14.60* | 28.91* | 17.44* | 28.10* | 63.27* | |
10-20 cm | PNV | 4.83±0.18b | 0.56±0.59a | 0.06±0.01b | 0.28±0.12c | 0.15±0.05b | 0.59±0.09b | 5.41±1.35a | 0.49±0.18b | 5.91±1.44a | 8.39±2.85c | 55.90±10.8b |
DNV | 4.70±0.15b | 0.11±0.18b | 0.02±0.01d | 0.07±0.03d | 0.04±0.01c | 0.66±0.09a | 4.36±1.25b | 0.14±0.05c | 4.50±1.24b | 3.51±1.79d | 82.51±5.32a | |
CCC | 4.93±0.29a | 0.90±0.59a | 0.05±0.01c | 0.64±0.33a | 0.17±0.05b | 0.48±0.10c | 4.17±1.09b | 0.86±0.38a | 5.03±1.13b | 17.61±8.96a | 38.08±13.08c | |
EAC | 5.04±0.43a | 0.79±0.52a | 0.08±0.01a | 0.45±0.32b | 0.25±0.05a | 0.51±0.17c | 5.15±1.37a | 0.79±0.51a | 5.95±1.63a | 13.11±6.17b | 43.17±18.09c | |
Mean | 4.88±0.26 | 0.59±0.47 | 0.05±0.01 | 0.35±0.20 | 0.15±0.04 | 0.56±0.11 | 4.78±1.27 | 0.57±0.28 | 5.35±1.36 | 10.65±4.94 | 54.91±11.82 | |
F-test | 6.15* | 11.94* | 15.25* | 23.97* | 20.95* | 14.62* | 6.13* | 22.45* | 7.32* | 25.41* | 60.69* |
Exchangeable bases (Ca2+, Mg2+ and K+) had the highest levels in the EAC area at all soil depths, with the exception of Ca2+ in the 10-20 cm depth layer, where the highest content was found in the CCC area. These results reflected in the increment of SB, CECp and V of the soil (Tab. 3). The soil of the DNV area presented high deficiency of exchangeable bases and the PNV area was naturally poor in nutrients. The areas under cultivation (CCC and EAC) had the highest P levels in the soil, but in subsoil they were not different from the PNV area (Tab. 3).
In general, we found low soil fertility in all the different land uses. The exchangeable bases, available P and pH were low, and Al saturation was high. CCC or EAC contributed to raise fertility levels and reduce acidity indicators, but the CECp of the PNV area was higher than the areas of cultivation (CCC and EAC). Further, the inappropriate exploitation of the DNV area degraded the soil, reducing its fertility and increasing acidity.
Total organic carbon and humic fractions of organic matter
Deforestation and different land use affected TOC (all depths) and the humic fractions of organic matter HA-C (all depths), HU-C at 0-5 cm depth and HS-C at 0-5 cm and 10-20 cm depths (Tab. 4). The PNV and EAC areas presented the highest levels of TOC in the superficial layer. Reduction of TOC in the CCC and DNV areas was of 10% and 20%, respectively, in relation to the PNV area. In the subsurface layers (5-10 and 10-20 cm), the CCC area accumulated more organic C and TOC contents reached the same levels of PNV and EAC areas (Tab. 4).
Tab. 4 - Total organic carbon and humic fractions of the organic matter of topsoil (0-5 cm) and subsoil (5-10 and 10-20 cm) under forest, deforested, cropland and agro-energy. (PNV): preserved native vegetation; (DNV): degraded native vegetation; (CCC): Cassava conventional cultivation; (EAC): Eucalyptus agro-energy cultivation. (TOC): total organic carbon; (HA-C): humic acid fraction; (FA-C): fulvic acid fraction; (HU-C): humin fraction; (HS-C): humic substances fractions; (HA-C/FA-C): humic acid fraction/fulvic acid fraction; (HS-C/TOC): humic substances fractions/total organic carbon. Mean values and standard deviation are reported. Values in columns with similar letter are not significantly different (p>0.05). (ns): not significant.
Soil Depth |
Site | TOC (g kg-1) |
HA-C (g kg-1) |
FA-C (g kg-1) |
HU-C (g kg-1) |
HS-C (g kg-1) |
HA-C/FA-C (g kg-1) |
HS-C/TOC (g kg-1) |
---|---|---|---|---|---|---|---|---|
0-5 cm | PNV | 14.16 ± 2.23 a | 3.21 ± 1.73 a | 2.82 ± 2.05 a | 6.34 ± 1.73 a | 12.37 ± 2.88 a | 1.14 ± 0.51 a | 0.87 ± 0.26 a |
DNV | 11.26 ± 3.00 c | 1.97 ± 1.43 b | 2.56 ± 1.59 a | 4.81 ± 2.73 b | 9.34 ± 3.55 b | 0.77 ± 0.34 b | 0.83 ± 0.39 a | |
CCC | 12.78 ± 2.56 b | 2.33 ± 1.75 b | 2.88 ± 1.72 a | 5.67 ± 1.80 a | 10.88 ± 3.06 b | 0.81 ± 0.36 b | 0.85 ± 0.30 a | |
EAC | 14.31 ± 2.72 a | 3.67 ± 2.47 a | 2.84 ± 1.28 a | 5.75 ± 2.01 a | 12.26 ± 3.48 a | 1.29 ± 0.58 a | 0.86 ± 0.28 a | |
Mean | 13.13 ± 2.63 | 2.80 ± 1.85 | 2.78 ± 1.66 | 5.64 ± 2.15 | 11.21 ± 3.24 | 1.00 ± 0.45 | 0.85 ± 0.31 | |
F-test | 7.70* | 3.93* | 0.62 ns | 2.83* | 5.30* | 1.67* | 0.38 ns | |
5-10 cm | PNV | 12.17 ± 2.19 a | 3.06 ± 0.82 a | 2.92 ± 0.95 a | 3.59 ± 2.23 a | 9.57 ± 2.96 a | 1.04 ± 0.68 a | 0.79 ± 0.36 a |
DNV | 9.48 ± 1.70 b | 1.93 ± 0.52 b | 2.51 ± 0.73 a | 3.11 ± 1.93 a | 7.55 ± 2.84 a | 0.77 ± 0.80 b | 0.80 ± 0.41 a | |
CCC | 11.16 ± 2.00 a | 2.04 ± 0.55 b | 2.83 ± 0.97 a | 3.19 ± 1.79 a | 8.06 ± 2.66 a | 0.72 ± 0.54 b | 0.72 ± 0.34 a | |
EAC | 12.00 ± 2.16 a | 3.07 ± 0.83 a | 3.05 ± 1.13 a | 3.23 ± 2.24 a | 9.35 ± 4.62 a | 1.01 ± 0.67 a | 0.78 ± 0.36 a | |
Mean | 11.20 ± 2.05 | 2.52 ± 0.68 | 2.83 ± 0.95 | 3.28 ± 2.05 | 8.63 ± 3.27 | 0.88 ± 0.67 | 0.77 ± 0.37 | |
F-test | 2.49* | 2.97* | 1.45 ns | 0.26 ns | 2.18 ns | 2.31* | 0.19 ns | |
10-20 cm | PNV | 7.73 ± 2.08 a | 1.90 ± 1.25 a | 2.85 ± 1.38 a | 1.98 ± 0.95 a | 6.73 ± 2.21 a | 0.67 ± 0.51 a | 0.87 ± 0.35 a |
DNV | 6.03 ± 1.28 b | 0.66 ± 0.80 b | 2.20 ± 1.73 a | 1.81 ± 0.89 a | 4.67 ± 2.01 b | 0.30 ± 0.44 b | 0.77 ± 0.36 a | |
CCC | 7.49 ± 2.07 a | 1.11 ± 0.75 b | 2.61 ± 1.53 a | 1.83 ± 0.84 a | 5.55 ± 1.74 b | 0.43 ± 0.86 b | 0.74 ± 0.41 a | |
EAC | 7.61 ± 1.73 a | 1.24 ± 0.94 b | 2.89 ± 2.22 a | 1.86 ± 1.02 a | 5.99 ± 2.40 a | 0.43 ± 0.44 b | 0.79 ± 0.33 a | |
Mean | 7.21 ± 1.79 | 1.23 ± 0.94 | 2.64 ± 1.72 | 1.87 ± 0.93 | 5.74 ± 2.09 | 0.45 ± 0.56 | 0.79 ± 0.36 | |
F-test | 5.24* | 7.89* | 0.93 ns | 0.19 ns | 5.13* | 1.82* | 0.61 ns |
The HA-C fractions in the PNV and EAC areas were also higher than in the CCC and DNV areas in the superficial layers (0-5 and 5-10 cm), but the PNV area had the highest C content in the HA-C fraction in the subsurface layer (10-20 cm). The C of the HU-C fraction in the CCC area showed a reduction of 24% in relation to the more preserved area (PNV) at 0-5 cm depth. In the CCC and DNV areas, there was a reduction of the C content on the HS-C fraction in layers of 0-5 cm and 10-20 cm depth (Tab. 4).
In general, a low level of humification (<45%) was observed in the soil of all land uses, as organic C of the HU-C fraction represented on average 43%, 29% and 26% of TOC at 0-5 cm, 5-10 cm and 10-20 cm depths, respectively (Tab. 4). However, the PNV plot showed the highest level of humification (45%) in the surface layer.
TOC reduction with depth ranged from 41% to 47% between the 0-5 cm and 10-20 cm depth layers, with the EAC area showing the greatest reduction (Tab. 4). The litter produced by eucalyptus, concentrating more organic C on the soil surface. The HS-C fraction was reduced in depth by 46%, 50%, 49% and 51% in the areas of PNV, DNV, CCC and EAC, respectively (Tab. 4). The HA-C fraction was reduced with depth approximately 1.5-fold more in the DNV area than in the PNV area (Tab. 4). The stability of the organic C in the DNV area was higher in the superficial layer and in the PNV area the distribution of the more stable fraction of the organic C (HA-C) was more homogeneous. The HA-C/FA-C ratio was higher than 1 in the superficial layers of soil of the PNV and EAC areas (Tab. 4), showing a predominance of humic acids over fulvic acids and confirming the greater stability of SOM.
The first two components of the PCA carried out on soil chemical properties accounted for 96% of the total variation in the 0-5 cm depth layer. PC1 was associated with most of the chemical properties (pH, P, K+, Ca2+, Mg2+, Al3+, SB and Al saturation) and all humic fractions of SOM (TOC, HA-C, FA-C, HU-C, HS-C and HA-C/FA-C), with the exception of HS-C/TOC, while PC2 was associated with properties (H+Al) and V (Tab. 5).
Tab. 5 - Correlation between each principal component (PC) and soil chemical attributes and humic fractions of the organic matter in topsoil (0-5 cm) and subsoil (5-10 cm and 10-20 cm). (pH): hydrogen potential (H2O/1:2.5); (P): available phosphorus; (K+): exchangeable potassium; (Ca2+): exchangeable calcium; (Mg2+): exchangeable magnesium; (Al3+): exchangeable aluminum; (H+Al): potential Acidity; (SB): sum of bases; (CECp): cations exchange capacity potential; (V): base saturation; (Al saturation): saturation by aluminum; (TOC): total organic carbon; (HA-C): humic acid fraction; (FA-C): fulvic acid fraction; (HU-C): humin fraction; (HS-C): humic substances fractions; (HA-C/FA-C): humic acid fraction/fulvic acid fraction; (HS-C/TOC): humic substances fractions/total organic carbon. (*): p<0.05.
Attribute | 0-5 cm | 5-10 cm | 10-20 cm | |||
---|---|---|---|---|---|---|
PC1 | PC2 | PC1 | PC2 | PC1 | PC2 | |
pH | 0.931* | -0.325 | 0.847* | -0.508 | 0.870* | -0.393 |
P | 0.794* | -0.606 | 0.703* | -0.671 | 0.887* | -0.430 |
K+ | 0.941* | 0.332 | 0.949* | 0.151 | 0.881* | 0.192 |
Ca2+ | 0.832* | -0.509 | 0.852* | -0.473 | 0.746* | -0.614 |
Mg2+ | 0.960* | -0.195 | 0.934* | -0.288 | 0.935* | -0.204 |
Al3+ | -0.879* | 0.454 | -0.727* | 0.687 | -0.798* | 0.592 |
(H+Al) | 0.410 | 0.884* | 0.563 | 0.701* | 0.553 | 0.766* |
SB | 0.904* | -0.416 | 0.914* | -0.400 | 0.858* | -0.506 |
CECp | 0.694 | 0.677 | 0.742* | 0.606 | 0.875* | 0.421 |
Al saturation | -0.890* | 0.370 | -0.865* | 0.469 | -0.903* | 0.402 |
V | 0.659 | -0.737* | 0.718* | -0.696 | 0.733* | -0.633 |
TOC | 0.954* | 0.294 | 0.936* | 0.340 | 0.981* | 0.094 |
HA-C | 0.924* | 0.277 | 0.819* | 0.532 | 0.717* | 0.645 |
FA-C | 0.952* | 0.233 | 0.999* | 0.010 | 0.966* | 0.236 |
HU-C | 0.798* | 0.587 | 0.498 | 0.750* | 0.513 | 0.826* |
HS-C | 0.921* | 0.386 | 0.861* | 0.506 | 0.826* | 0.545 |
HA-C /FA-C | 0.873* | 0.294 | 0.679 | 0.683 | 0.639 | 0.684 |
HS-C /TOC | 0.696 | 0.658 | 0.650 | 0.725* | 0.253 | 0.964* |
Absolute variance (%) | 71.60 | 24.40 | 65.20 | 29.30 | 63.30 | 31.00 |
Cumulated variance (%) | 71.60 | 96.00 | 65.20 | 94.50 | 63.30 | 94.30 |
Regarding the 5-10 cm depth layer, the first two components explained 94.5% (PC1: 65.20%, PC2: 29.30%) of the variance in soil chemical properties and humic fractions of SOM. With the exception of (H+Al), HU-C, HA-C/FA-C and HS-C/TOC, all other properties were correlated with PC1, while PC2 was associated with (H+Al), HU-C and HS-C/TOC (Tab. 5).
In the 10-20 cm depth layer, PC1 and PC2 were responsible for 94.30% of the total variance (63.30% and 31.00%, respectively). Soil chemical properties and the SOM fractions that most correlated with PC1 were: pH, P, K+, Ca2+, Mg2+, Al3+, SB, CECp, Al saturation, V, TOC, HA-C, FA-C and HS-C. The soil property (H + Al) and SOM fractions HU-C and HS-C/TOC were those showing the stronger association with PC2 (Tab. 5).
The acidity indicators Al3+ and Al saturation correlated negatively with pH, SB (except for K+), V and P in all soil layers (Fig. 4). In areas with a predominance of acidity, soil fertility was limited both by lower levels of exchangeable basic cations and by low soil P content. The CECp correlated positively with the contents of (H + Al), TOC and the humic fractions of SOM. The ability to adsorb cations in these environments was dependent on SOM, showing its influence on soil fertility (Fig. 4).
Fig. 4 - Biplots of the soil chemical properties and humic fractions of the organic matter. (a) topsoil (0-5 cm), (b) subsoil 1 (5-10 cm) and (c) subsoil 2 (10-20 cm). (PNV): preserved native vegetation; (DNV): degraded native vegetation; (CCC): Cassava conventional cultivation; (EAC): Eucalyptus agro-energy cultivation; (pH): hydrogen potential (H2O/1:2.5); (P): available phosphorus; (K): exchangeable potassium; (Ca): exchangeable calcium; (Mg): exchangeable magnesium; (Al): exchangeable aluminum; (H+Al): potential Acidity; (SB): sum of bases; (CECp): cations exchange capacity potential; (V): base saturation; (Al saturation): saturation by aluminum; (TOC): total organic carbon; (HA-C): humic acid fraction; (FA-C): fulvic acid fraction; (HU-C): humin fraction; (HS-C): humic substances fractions; (HA-C/FA-C): humic acid fraction/fulvic acid fraction; (HS-C/TOC): humic substances fractions/total organic carbon. The arrows represent the directions of the high weighting of soil chemical properties and humic fractions of the organic matter along the first (PC1) and second (PC2) principal components. PC1 and PC2 of topsoil explained 96.00% of the total variation, while they accounted for 94.50% and 94.30% of the variation is soil chemical parameters in subsoil 1 (5-10 cm) and subsoil 2 (10-20 cm), respectively.
The TOC and humic fractions of SOM were positively correlated. However, their correlation is stronger in the superficial layer and becomes weaker in depth, with TOC showing greater association with FA-C in the layer 10-20 cm. Moreover, the vector of the parameter HU-C in Fig. 4is less overlapping with that of TOC, indicating the low humification level of this TOC.
The chemical properties pH, P, Ca2+, Mg2+ and V reached their largest values in the soil of EAC and CCC plots. The PNV areas were associated with TOC and humic fractions of SOM. The DNV area was more influenced by acidic properties Al3+ and Al saturation (Fig. 4). The CCC soil properties approached those of the DNV area in the surface layer, while they are less similar as for deeper layers of the soil (Fig. 4).
The dendrogram of similarity between different land uses based on soil chemical properties (Fig. 5) showed that the DNV area differed from the other areas in all soil layers. The PNV area is similar to the cultivated areas in superficial layers (CCC, 0-5 cm and EAC, 5-10 cm).
Fig. 5 - Dendrograms of the different land uses according to the soil chemical properties and humic fractions of the organic matter. (a) topsoil (0-5 cm); (b) subsoil 1 (5-10 cm); (c) subsoil 2 (10-20 cm). (PNV): preserved native vegetation; (DNV): degraded native vegetation; (CCC): Cassava conventional cultivation; (EAC): Eucalyptus agro-energy cultivation.
Discussion
The soils of areas with different land uses showed comparable texture at the studied depths (Tab. 2). Many physical and chemical properties of the soil are directly affected by texture, such as bulk density, pore size distribution, CEC, SOM, and others. In view of this, the deforestation and land use changes significantly influenced their properties.
Soil physical properties
We found that the increase in BD in DNV was explained by reduction in soil porosity (mainly MaP), agreeing with the findings of Bottinelli et al. ([8]) and Chen et al. ([11]). According to Hebb et al. ([16]), the decrease of MaP, together with the increase of BD, decreases soil water permeability because drainage pores are main responsible for water percolation in the soil. Kuncoro et al. ([22]) found a positive correlation between water permeability and MaP, associating this result with the size and discontinuity of macropores.
The MaP little varied in the subsurface layer, indicating that different land uses weakly affect this physical property. Indeed, soil macroporosity may reflect the contribution of plant roots and plant residues, which occur mainly on the soil surface ([8]). In addition, we found a negative correlation between MaP and BD, which was generally higher in the subsurface layer, causing MaP reduction.
The degradation of soil physical properties in the DNV area can be favored by the reduced vegetation cover and the consequent higher exposure to rainfall ([3]) and animal trampling ([13]), which may cause the compaction of the surface layer. Taylor & Ashcroft ([45]) stated that values of MaP below 0.10 m3 m-3 limit gas exchange, impairing root respiration and development. In the PNV and EAC areas, the action of roots on the soil surface and larger increment of SOM may have promoted greater aggregation and structuring of the soil, as indicated by the higher values of MaP.
The highest values of BD and SRP in DNV were also related with the lower TOC content in this area in the 0-5 and 10-20 cm layers, promoting lower root content and microbial action as SOM improves structuring and porosity ([19]). SRP values greater than 2.0 MPa are above the critical levels reported by Taylor et al. ([44]), and this can hamper plant root growth and favor loss of water and air from soil pores.
The strong association between the MiP, FC and AW soil properties in the CCC area may be explained by the greater homogenization due to tillage applied for crop cultivation, thus reducing pores and increases water retention ([25]). However, the similarity between the EAC area in the subsurface layer and the DNV area may be due to a lower decomposition of plant residues in the subsurface ([51]), which results in low aggregation capacity of the soil in deeper layers and consequently in the degradation of the physical properties of the soil.
Soil chemical properties
The highest values of pH in cultivated areas (CCC and EAC) can be attributed to liming and fertilization practices that increased the number of exchangeable bases and soil pH ([1]). In these areas, also Al+3 complexation may occur due to SOM acid groups ([17]). In CCC area the plant residues are usually incorporated in the soil through tillage or hoeing, while in EAC area are maintained on the soil surface.
The high acidification of the DNV area may be related to the reduction of exchangeable bases due to surface erosion and leaching during periods of higher rainfall, as a consequence of the removal of native vegetation leaving the soil exposed ([27]). However, in areas of preserved native vegetation, soil pH is also usually acid ([6]). According to Silva et al. ([36]) in areas of preserved vegetation, the ecosystem in equilibrium tends to a natural acidification of the soil due to SOM mineralization and the release of acidic exudates by plant root systems.
The high amounts of exchangeable bases in the soil of planted forests such as eucalyptus cultivation may be associated with fertilization and liming practices in these areas and to the contribution of plant material to the soil surface. According to Monroe et al. ([28]), forested areas have the capacity to increase soil fertility by the addition of organic matter to soil from both the above- and belowground biomass.
The amount of exchangeable bases in areas of preserved vegetation are naturally low ([25]). Allocation of these nutrients to plant biomass and its return to the soil through the cycling process adequately nourishes the plants ([23]).
The high P availability in the soil of these dry ecosystems is due to chemical fertilization. However, in areas with preserved vegetation, it can be promoted by the organic material contribution, which reduces the adsorption and precipitation of P ([6]), besides being a source of release ([52]) and recycling of P in organic form ([28]).
Total organic carbon and humic fractions of organic matter
The similarity of TOC contents between PNV and EAC areas in the soil surface layer showed that increase in TOC content is favored by continuous deposition of litter and by the presence of more developed root systems on the surface ([10], [28]), which may contribute to maintain soil moisture and reduce soil erosion ([15]). In this study, the reduction of TOC in the DNV and CCC areas indicated that the soil preparation, which exposes SOM to weathering, and the lack of conservation practices in the CCC area have reduced physical protection of SOM and left the soil exposed to erosion ([27], [49]). These results corroborate with Sharma et al. ([34]) who found lower TOC levels in an environment of conventional agriculture and in degraded areas compared to those where the soil was kept covered.
The increase of HA-C in PNV and EAC areas reveals that the management that maintains soil coverage, adequate humidity and minimal or no anthropic action may promote microbial action and humic fraction production, and more developed root systems as well ([29], [43]). In fact, lower accumulation of HA-C in DNV and CCC areas may be related to the process of degradation of the areas due to soil exposure to erosive processes ([27]) and/or to the low increment of plant biomass.
The higher proportion of HU-C in relation to HA-C and FA-C in the topsoil of different land uses is due to its molecule size which determines a higher chemical affinity to colloids ([27]), making them more stable and resistant to weathering. However, the microbial activity is reduced in semi-arid regions ([39]), resulting in a low content of this fraction of SOM. Levels of HU-C similar to those of this study were found by Sousa et al. ([39]) and Melo et al. ([27]) who investigated the behavior of the different SOM fractions in soils of the Brazilian semi-arid region.
Low biomass production and low organic matter input to soil are common in semi-arid regions ([27]). However, the HA-C/FA-C ratio was > 1 in the PNV and EAC areas due to the greater contribution of organic material, reflecting the high degree of humification in these soils ([10]). Contrastingly, the HA-C/FA-C ratio < 1 in DNV and CCC areas suggests a lower contribution of organic residues and lower microbial activity, which hampers the formation of more condensed humic substances ([27]). The HA-C/FA-C ration indicates the quality of humus of the soil, with smaller ratios expected for soils subjected to more intense degradation processes ([41]).
The humification degree (HS-C/TOC) varying from 72 to 87% in all areas under different land uses indicates that most of TOC is in humic fractions, suggesting a high level of humification.
We found that P, Ca2+ and TOC, SB and Al saturation were the most important chemical properties to explain data variation, in addition to the HA-C fraction of SOM, which strongly discriminate areas under different land uses. Marinho et al. ([24]) and Sousa Neto et al. ([40]), studying different management systems in the semi-arid region of Brazil, found that the different sites could be discriminated based on soil chemical properties such as pH, P, K+, Ca2+, Mg2+, CEC and TOC.
The negative correlation of Al3+ with pH, nutrient availability and humic fractions of SOM suggests a complexation of Al3+ by humic substances ([47]), resulting in an increase of nutrient availability and soil pH. In fact, our results highlight the influence of organic matter on soil fertility as a source of nutrients for the plants, with K+ being the most mineralized nutrient in the study areas. The relationship between CEC and TOC showed that a higher organic content in the soil can be very important in the generation of electric charges, maximizing the soil’s ability to retain cations ([47]).
The close association of FA-C with TOC in the subsurface may be due to characteristics of the FA, which is highly soluble and mobile in the soil, and thus its content incraeses with depth ([33]). Contrastingly, we observed a greater distance between the HU-C and TOC vectors, suggesting a small influence of this SOM fraction on deeper layers of the soil.
We found that the differences in soil chemical properties between DNV and the other land uses become weaker in the subsurface layer, and this may reflect the low capacity of the different land uses to improve chemical quality and SOM at the lower soil depths in these dry habitats. The higher recalcitrance of the eucalyptus plant material ([51]), associated with the water conditions of the semi-arid region ([27]) may explain these results.
The clustering of CCC and EAC areas in soil subsurface indicated that cultivation practices adopted in these areas did not affect the soil chemical properties and SOM fractions. The low capacity of eucalyptus to improve soil conditions at lower depth ([51]) may explain the above similarity, mainly in terms of TOC and SOM fractions. Further, the independent clustering of DNV from the other land uses may be due to the strong degradation of the soil quality resulting from lower vegetation cover, greater erosion and leaching, and lower input of organic material.
SOM preservation and transformation processes in different land uses has the greatest importance and has to be studied in detail. However, it has been widely questioned whether the traditional chemical fractionation involving extraction with strong alkali can provide information on SOM dynamics and turnover in soils ([20]). The alkali extraction is often considered less suitable to obtain information on SOM preservation and turnover than physical fractionation methods, such as density fractionation procedures ([21]), which can distinguish light active fractions, occluded intermediate fractions and mineral-associated, more stable C pools. Nonetheless, the chemical fractionation method used in this study provided good indicators of the SOM preservation and transformation processes.
Finally, the improvement of soil properties in the surface layer by the EAC does not prove the hypothesis tested.
Conclusions
The physical and chemical properties that best described the soil quality of different land uses in Chapada do Araripe were MaP, BD, SRP, SB (mainly Ca2+), available P and Al saturation. The TOC and the HA-C fraction of SOM were also important in improving soil quality. The DNV area showed degradation of both chemicals and physical properties, resulting in the reduction of soil quality. Improvement of these properties in the EAC area was limited to the surface layer. Grouping obtained by cluster analysis showed that DNV (degraded native vegetation) and PNV (preserved native vegetation) areas are different from the cultivated areas (EAC and CCC) in terms of soil physical and chemical parameters, though EAC and CCC areas approach the PNV area in the soil surface layers, while DNV showed a strong reduction in soil quality.
Our study suggests that the disordered cutting of native vegetation causes land degradation in Chapada do Araripe, which results in the reduction of soil quality. Crops that allow the input of biomass and keep the soil covered, protecting it from weathering, can maintain or improve soil properties and functions, although this improvement has not been observed below the soil surface (>10 cm depth).
Author contributions
JSR: investigation, methodology, formal analysis and writing the original draft; FJF; conceptualization, funding acquisition, supervision, writing review and editing; JCAF: resources, methodology and data curation; MBGSF: resources, methodology and visualization; BGA: formal analysis, data curation and visualization; LRCS: formal analysis and data curation.
Acknowledgements
The authors thank the Experimental Station of Araripina of the Agronomic Institute of Pernambuco (IPA), Brazil for the logistic and operational support. The study was supported by Pernambuco State Science and Technology Support Foundation (FACEPE) and by the National Council for Scientific and Technological Development (CNPq), project no. APQ-0729-5.01/14 “Energia de biomassa em Pernambuco: impactos ambientais e alternativas sustentáveis (ProMassa)”.
References
CrossRef | Gscholar
Gscholar
Gscholar
Online | Gscholar
Gscholar
Gscholar
Gscholar
Authors’ Info
Authors’ Affiliation
Universidade Estadual do Piauí, Campus Prof. Barros Araújo, Rodovia BR-316, KM 299, Altamira 64602000 Picos, PI (Brazil)
Maria Betânia Galvão Dos Santos Freire 0000-0002-0872-5909
Brivaldo Gomes de Almeida 0000-0003-3260-0767
Leandro Reis Costa Santos
Universidade Federal Rural de Pernambuco, Rua Dom Manoel de Medeiros, s/n, Dois Irmãos, 52171-900 Recife, PE (Brazil)
Empresa Brasileira de Pesquisa Agropecuária (EMBRAPA), Rua Antônio Falcão, 402, Boa Viagem 51020-240 Recife, PE (Brazil)
Corresponding author
Paper Info
Citation
Souza Rezende J, Freire FJ, Araújo Filho JC, Dos Santos Freire MBG, Gomes de Almeida B, Costa Santos LR (2022). Impact of deforestation on the soil physical and chemical attributes, and humic fraction of organic matter in dry environments in Brazil. iForest 15: 465-475. - doi: 10.3832/ifor4016-015
Academic Editor
Giorgio Alberti
Paper history
Received: Nov 10, 2021
Accepted: Sep 12, 2022
First online: Nov 18, 2022
Publication Date: Dec 31, 2022
Publication Time: 2.23 months
Copyright Information
© SISEF - The Italian Society of Silviculture and Forest Ecology 2022
Open Access
This article is distributed under the terms of the Creative Commons Attribution-Non Commercial 4.0 International (https://creativecommons.org/licenses/by-nc/4.0/), which permits unrestricted use, distribution, and reproduction in any medium, provided you give appropriate credit to the original author(s) and the source, provide a link to the Creative Commons license, and indicate if changes were made.
Web Metrics
Breakdown by View Type
Article Usage
Total Article Views: 27660
(from publication date up to now)
Breakdown by View Type
HTML Page Views: 21947
Abstract Page Views: 3791
PDF Downloads: 1563
Citation/Reference Downloads: 4
XML Downloads: 355
Web Metrics
Days since publication: 958
Overall contacts: 27660
Avg. contacts per week: 202.11
Article Citations
Article citations are based on data periodically collected from the Clarivate Web of Science web site
(last update: Mar 2025)
Total number of cites (since 2022): 3
Average cites per year: 0.75
Publication Metrics
by Dimensions ©
Articles citing this article
List of the papers citing this article based on CrossRef Cited-by.
Related Contents
iForest Similar Articles
Research Articles
Soil fauna communities and microbial activities response to litter and soil properties under degraded and restored forests of Hyrcania
vol. 14, pp. 490-498 (online: 11 November 2021)
Research Articles
Effects of tree species, stand age and land-use change on soil carbon and nitrogen stock rates in northwestern Turkey
vol. 9, pp. 165-170 (online: 18 June 2015)
Research Articles
Wood-soil interactions in soil bioengineering slope stabilization works
vol. 2, pp. 187-191 (online: 15 October 2009)
Research Articles
Soil stoichiometry modulates effects of shrub encroachment on soil carbon concentration and stock in a subalpine grassland
vol. 13, pp. 65-72 (online: 07 February 2020)
Research Articles
Comparison of soil CO2 emissions between short-rotation coppice poplar stands and arable lands
vol. 11, pp. 199-205 (online: 01 March 2018)
Research Articles
Soil respiration along an altitudinal gradient in a subalpine secondary forest in China
vol. 8, pp. 526-532 (online: 01 December 2014)
Review Papers
Soil fungal communities across land use types
vol. 13, pp. 548-558 (online: 23 November 2020)
Research Articles
The manipulation of aboveground litter input affects soil CO2 efflux in a subtropical liquidambar forest in China
vol. 12, pp. 181-186 (online: 10 April 2019)
Research Articles
Effects on soil characteristics by different management regimes with root sucker generated hybrid aspen (Populus tremula L. × P. tremuloides Michx.) on abandoned agricultural land
vol. 11, pp. 619-627 (online: 04 October 2018)
Research Articles
Estimating changes in soil organic carbon storage due to land use changes using a modified calculation method
vol. 8, pp. 45-52 (online: 17 June 2014)
iForest Database Search
Search By Author
Search By Keyword
Google Scholar Search
Citing Articles
Search By Author
Search By Keywords
PubMed Search
Search By Author
Search By Keyword