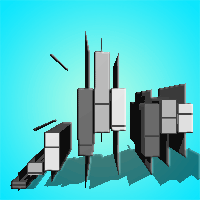
Growth, morphology, and biomass allocation of recently planted seedlings of seven European tree species along a light gradient
iForest - Biogeosciences and Forestry, Volume 13, Issue 4, Pages 261-269 (2020)
doi: https://doi.org/10.3832/ifor3370-013
Published: Jul 03, 2020 - Copyright © 2020 SISEF
Research Articles
Abstract
Light is one of the most critical factors controlling tree survival and growth. Limited light availability induces phenotypic plasticity, thus enabling plants to adapt to suboptimal conditions. The plastic responses are species-specific and are thought to largely depend on species’ shade tolerance. This study aims to add to existing research by trying to disentangle the effects of light, species identity, and shade tolerance on growth, biomass partitioning, and morphology of seedlings of seven common European tree species. For that purpose, we set up a shading experiment where seedlings were grown under three levels of light availability (15%, 35%, and 100%). A destructive harvest was carried out for the assessment of biomass allocation and structural complexity of plant architecture after a year of exposure to limited light. The specific leaf area increased with decreasing light availability for all species. However, we found little to no changes in relative height and diameter growth, biomass allocation to aboveground tree compartments, and structural complexity along the light gradient. We argue that because trees were grown under open field conditions, both in the nursery and for the first year of the experiment, it might have resulted in a delayed response to limited light availability. Assuming the delayed reaction of less plastic plant organs, we expect that the morphological adaptations of the tree species and intra- and interspecific differences will become more pronounced, as the trees grow older.
Keywords
Shade Tolerance, Plant Morphology, Fractal Analysis, Biomass Allocation, Specific Leaf Area, Light
Introduction
Plant phenotypic plasticity is the natural consequence of their immobility ([4]). Different biotic (e.g., competition, herbivory) and abiotic (e.g., light, temperature, soil conditions, water availability) environmental factors affect plant growth and induce phenotypic plasticity. The latter reveals itself in the form of changes in plant morphology, biomass allocation patterns, or differences in the structural complexity of plant architecture ([27]). Generally speaking, the plasticity of physiological or morphological traits is a beneficial characteristic as it enables a plant to adapt to suboptimal environmental conditions ([5]). Understanding the rates and expressions of the plasticity of various species and their relation to shade tolerance can help to improve the management of mixed forests, including continuous cover forestry.
Among the influential factors for phenotypic plasticity, light availability is recognized as one of the most important drivers, strongly controlling tree survival and growth ([25], [24], [8]). Additionally, the net competitive ability (i.e., strategical growth response as a result of resource limitation) is found to be different between species ([20]). One of the most important determinants of these differences is species’ shade tolerance. Shade tolerance is, to a large extent, dependent on the plasticity of light-harvesting organs ([42]), and is often quantified either as the ability to survive or as the rate of growth when a given resource is extremely limited ([46]). However, shade tolerance is a complex and specific adaptation ([16]). Some authors even argue that it should not be perceived as an absolute value, but rather a relative concept that depends on specific ecological context (e.g., a co-existence of multiple biotic and abiotic stressors) and ontogenic stage ([43]). Although species shade tolerance has been widely investigated, there still appears to be a lack of consensus regarding the relevant traits through which species adapt to low-light environments ([43]).
Delagrange et al. ([10]) studied the physiological, morphological, and allocational plasticity of trees under different light levels. They concluded that single traits such as leaf area ratio, maximal stomatal conductance, crown height to crown diameter ratio, or leaf nitrogen concentration cannot explain the interspecific differences in shade tolerance. Hence, the concept of shade tolerance remains a rather qualitative one, and the question of what shade tolerance specifically is still prevails. Is it only related to species’ ability to survive in low-light environments, or does the concept encompass interactions between fluctuations in environmental conditions and a plant’s phenotypic plasticity? Understanding the light requirements of different species allows creating favorable conditions for desired mixtures and ensuring more successful regeneration practices ([26]). Therefore, further in-depth studies are required to test the regulatory mechanisms on multiple levels, i.e., morphological, anatomical, physiological, and molecular.
Resource availability affects growth ([14]), biomass allocation ([6]), and the structural complexity of plants ([2]). For most species, height growth, diameter growth, and overall biomass production are positively related to increased light availability ([24], [23], [39]). However, the growth strategies of plants differ and are dependent on species identity ([1]). Shade-tolerant species are expected to grow slower and use the available resources more conservatively, whereas light-demanding species are expected to exhibit higher growth rates and avoid shade when the light availability is limited ([31]). These differences persist both in low- and high-light environments because of the higher photosynthetic plasticity of light-demanding species ([30]). Higher photosynthetic plasticity allows plants to use light more efficiently, even if the light availability is limited ([42]).
Resource availability also affects the allocational plasticity of plants. Biomass allocation varies between species, environments, and over time ([28]). Moreover, according to the balanced growth hypothesis ([40]), plants are expected to allocate more biomass towards the organ that is responsible for acquiring the limited resource. For example, in case of limited light availability, plants will allocate more biomass towards the aboveground ([19]), preferably to leaves or needles ([29]).
Leaves and needles are considered the most plastic organs, and in low-light conditions, trees firstly tend to adapt by changing their leaf morphology ([28]). Against this background, specific leaf area (SLA) is typically found to increase with decreasing light availability ([24], [33], [18]). Moreover, SLA of shade-tolerant species is usually higher than of light-demanding ones ([12], [26], [39]).
Changes in allocation patterns also translate into changed structural complexity, e.g., light-demanding species tend to invest more in leaf area and stem elongation ([48]), thus directly influencing the structural complexity of the plant architecture. The structural complexity of a plant directly relates to how the plant occupies space and intercepts light ([32]). Accordingly, plants are expected to form structurally more complex branching patterns when the light availability is not limited ([2], [37]). The box-dimension values derived from fractal analysis can be used to assess structural complexity of plant architecture ([22], [36]). Increased structural complexity translates into higher box-dimension values that are derived from the number of different-sized voxels fit to a 3D tree model ([36]).
A plethora of studies has analyzed the influence of light availability on tree growth and biomass allocation, but the findings are still divergent. Species’ shade tolerance rankings, in terms of growth and biomass allocation, are mostly consistent ([18], [44]). However, numerous studies found responses to light in the expression of one or more traits that did not follow traditional shade tolerance rankings ([7], [11]).
This paper presents the results from the first two years of a long-term experiment studying seedlings of seven common European tree species growing along a light gradient. The study seeks to add to the existing research by trying to disentangle the effects of light, species identity, and shade tolerance on growth, biomass partitioning, and structural complexity of common hornbeam (Carpinus betulus L.), European beech (Fagus sylvatica L.), Norway spruce (Picea abies [L.] H. Karst.), Scots pine (Pinus sylvestris L.), sessile oak (Quercus petraea [Matt.] Liebl.), silver fir (Abies alba Mill.), and sycamore maple (Acer pseudoplatanus L.). Additionally, it attempts to test the shade tolerance rankings introduced by Ellenberg & Leuschner ([13]), according to which the studied species can be arranged in the following order of decreasing shade tolerance: F. sylvatica (3) = A. alba (3) > C. betulus (4) = A. pseudoplatanus (4) > P. abies (5) > Q. petrea (6) > P. sylvestris (7).
The following hypotheses were tested:
- Height and diameter growth: (1.1) relative height and diameter growth differ between species; (1.2) relative height and diameter growth increase with increasing light availability; (1.3) relative height and diameter growth increase more strongly for light-demanding species.
- Biomass production and allocation: (2.1) total biomass increases with increasing light availability and the increase is stronger for light-demanding species; (2.2) root mass fraction (RMF) increases and leaf mass fraction (LMF) decreases with increasing light availability; (2.3) branch mass fraction (BMF) increases with increasing light availability for light-demanding species but remains constant for shade-tolerant species.
- Leaf and plant morphology: (3.1) SLA increases with decreasing light availability; (3.2) shade-tolerant species have higher SLA; (3.3) structural complexity increases with increasing light availability; (3.4) the structural complexity of light-demanding species increases more with increasing light availability.
Materials and methods
Study site and experimental setup
This study was conducted in a controlled shading experiment located at the North Campus of the University of Göttingen, Germany (51° 33′ 34.5″ N, 09° 57′ 34.8″ E). The study site is located 234 m above sea level. According to Köppen-Geiger climate classification, the climate is temperate and oceanic. The average annual temperature is 9.2 °C, and the annual average precipitation reaches 650 mm, of which 310 mm falls between May to September (⇒ http://www.dwd.de/).
Eighty centimeters (80 cm) of topsoil were dug out, mixed with homogeneous sand (2:1), and filled back in up to the ground level to create homogeneous growth conditions. The soil was covered with a fabric sheet of woven weed control membrane to avoid the emergence of competing vegetation. An automatic dripping irrigation system was installed, and all seedlings received the same water treatment. The soil was kept moist so that light was the only limited resource. Precipitation was able to reach the seedlings, thus providing additional water.
The shading experiment was established for seven common European tree species, i.e., F. sylvatica, A. alba, A. pseudoplatanus, C. betulus, P. abies, Q. petrea, and P. sylvestris.
The planting material was obtained from August Lüdemann Forst und Landschaftservice GmbH (Frankfurt, Germany). P. sylvestris seedlings were one year old and the seedlings of all the other species - two-year-old. The seedlings were not transplanted or undercut. All seedlings were bareroot and their initial size ranged from 30-50 cm, on average.
The seedlings were planted in November 2016. During the first year, plants grew under open field conditions to avoid high initial mortality of the light-demanding species. In the second year, shading nets of different light permeability were installed on metal frames (height: 8 m) to create two additional light availability levels of 35% and 15% of the ambient light. The light levels were chosen based on the findings in literature ([46], [11]). It was aimed to expose seedlings to low enough light availability (15%) to study shifts in biomass allocation patterns, while avoiding high mortality of light-demanding species in long-term. The intermediate light level (35%) was chosen to represent good growth conditions for shade-tolerant species, some of which already saturate in growth above the given level (e.g., beech - [9]). Ambient light (100%) conditions we provided as the other extreme of unlimited light availability.
Irradiance was measured in every compartment both in an open field (control) and under the shading nets using the LI-COR QUANTUM® sensor (LI-COR Biosciences, Nebraska, USA) to control for the specified shading effect of the nets. Light availability was expressed as the percentage of irradiance passing through the shading nets in comparison to open field conditions.
The experiment was arranged in two levels - by light availability and by subplot (Fig. 1). Each of the three light availability levels (15%, 35%, and 100%) was assigned randomly to different shading halls. Subplots (Subplot 1 - Subplot 5) were used to categorize the experimental units by the time of the planned biannual seedling harvests, ranging from 2-10 years after planting. Seedlings were provided with enough growing space to insure that even after 10 years the competition would be excluded. Thus, seedlings in Subplot 1 (harvested for this study) each occupied 0.36 m2. The growing space for seedlings that will be harvested after 4, 6, 8, and 10 years was 0.71 m2, 1.43 m2, 3.21 m2, and 5.71 m2 per seedling, respectively. Against that background, the planting distances and the number of rows also varied by the subplot, (Subplot 1 and 2: 1 row, 0.7 m; Subplot 3: 2 rows, 1.0 × 1.4 m; Subplot 4: 3 rows, 1.2 × 2.2 m; Subplot 5: 4 rows, 1.6 × 2.5 m - Fig. 1). Each experimental unit (“n% light” × “Subplot n”, n=60) was comprised of 14 seedlings - two of each of the seven species - and replicated four times. The seedlings were planted in a random order within each experimental unit. In total, 120 seedlings per species were planted, i.e., 40 seedlings per light availability level.
Fig. 1 - The layout of the shading experiment. The experiment is arranged in two levels - by light availability and by subplot. There are three light availability levels (15%, 35%, and 100%), each replicated four times and assigned randomly to different shading halls. Additionally, the Subplot 1 - Subplot 5 are used to categorize the experimental units by the time of the planned biannual seedling harvests, ranging from 2-10 years after planting. Each experimental unit (“n% light” × “Subplot n”, n=60) is comprised of 14 seedlings - two of each of the seven tree species - and replicated four times. Planting distances and the number of rows vary by the subplot to provide enough space for seedlings that will have to grow for a longer period (Subplot 1 and 2 : 1 row, 0.7 × 0.7 m; Subplot 3: 2 rows, 1.0 × 1.4 m; Subplot 4: 3 rows, 1.2 × 2.2 m; Subplot 5: 4 rows, 1.6 × 2.5 m). The seedlings of different species are planted in a random order within each experimental unit. In total, 120 seedlings per species were planted, i.e., 40 seedlings per light availability level.
Sampling procedure
The height and diameter of each seedling were measured for two years at the end of the vegetation period. Height was measured using a folding ruler (Accuracy Class III). Diameter was measured as root collar diameter 5 cm above ground using a digital caliper (RS PRO 150mm Digital Caliper, accuracy ± 0.03 mm). Height and diameter measurement data from all seedlings were included in the data analysis (5 subplots × 3 light levels × 7 species × 8 seedlings = 840).
Destructive harvest was carried out for a subset (Subplot 1 - see Fig. 1) of 168 seedlings (3 light levels × 7 species × 8 seedlings = 168) at the end of the second vegetation period after planting, in November 2018. Leaves of the deciduous seedlings were harvested before the seedling harvest. Whereas for coniferous seedlings, the removal of needles took place in the lab after the whole-seedling harvest. A representative sample of 20 leaves or needles was collected and scanned for the leaf area estimates with an office-type scanner Medion MD 90093® (MEDION, Essen, Germany). The remaining leaves and needles were also removed, dried to constant weight, and weighted.
After the harvest and leaf or needle removal, all seedlings were scanned in the laboratory using a Faro Focus® 3D 120 (Faro Technologies, Lake Mary, FL, USA) terrestrial laser scanner. The seedlings were prepared for laser scanning by washing and consecutively air-drying for a short period, to avoid increased laser beam reflection when beams hit drops of water. Seedlings were mounted on a support stand with a two-prong clamp. Seedlings were adjusted so that the roots were hanging in the air and did not touch any objects. The root collar was in the middle of the prongs to provide a clear marker in the scan data. All seedlings were scanned using a multi-scan approach at four fixed scanning points at equal distance from the seedling according to the corners setup introduced in Van Der Zande et al. ([45]) but without the center scan.
After scanning, seedlings were partitioned into roots, stems, and branches and dried at 70 °C for at least 48 hours until a constant weight was reached. After drying, all the compartments were weighed. Leaf/needle, branch, stem, and root dry mass were determined to the milligram using a standard laboratory scale Sartorius MC1 LC1200S®, accuracy ± 0.003 g (Sartorius AG, Göttingen, Germany).
Data processing
Leaf and needle area were determined by processing the scanned images with the software WinFolia 2004a (Régent Instruments Inc., Quebec, Canada). SLA (cm2 g-1) was calculated as the leaf area divided by the oven-dry leaf mass.
Relative height (cm cm-1) and diameter (mm mm-1) growth between years 2018 and 2017 and biomass fractions (g g-1) were calculated for a more detailed analysis of interspecific differences in growth and biomass allocation along the light gradient. Following formulas were used to calculate the specific leaf area (SLA, cm2 g-1 - eqn. 1), relative growth (Rel_hinc: relative height increment, cm cm-1 - eqn. 2; and Rel_dinc: relative diameter increment, mm mm-1 - eqn. 3), and biomass allocation (tCMF: tree compartment mass fraction, g g-1 - eqn. 4):
where LA is the leaf area (cm2), LMd is the oven-dry leaf mass (g), h18 is the height in November 2018 (cm), h17 is the height in November 2017 (cm), d18 is the diameter in November 2018 (mm), d17 is the diameter in November 2017 (mm), BM_tC is the oven-dry biomass of a tree compartment, i.e., leaves/needles, branches, stems or roots (g), BM_tot is the oven-dry total plant biomass (g).
The spatial registration of the scans was based on four chessboard-style targets ([35]) and was done automatically using the software Faro Scene® 7 (Faro Technologies, Lake Mary, Florida, USA). For easier handling of the scans, the resolution was reduced to 1/16 of the original resolution, thus keeping it high enough to carry out analysis but allowing easier and faster processing. All scans were filtered using the standard filter settings of Faro Scene and exported as xyz-files.
Open source 3D point cloud processing software Cloud Compare v. 2.10.1 (⇒ http://www.cloudcompare.org/) was used to further process the xyz-files. The seedlings were virtually cut out from the point cloud. They were divided in above and below ground compartments by cutting in the middle of the prong clamp. All points belonging to the prong clamp were manually removed from the point clouds. The occluded stem volume was calculated as the volume of a cylinder using the height of the clamp and the seedling diameter at the root collar. Stray points were removed by visually assessing the above- and belowground point clouds of the seedlings.
Homogenous spatial resolution for the single-tree point clouds was obtained by transforming the point clouds to regular spatial grids with equal distances (0.2 cm) between points, so-called point cloud grids, as introduced in Seidel et al. ([35]).
Above- and belowground box-dimensions (Db) were used as holistic measures of structural complexity of plant architecture ([22]). Box-dimensions were obtained for each tree individually using the point cloud grids of the seedlings. The box-dimension values describe the slope of the linear regression of the number of virtual boxes filled with plant material fitted against inverse values of box sizes used to cover the whole plant. All the values are logarithmically transformed, and the slope of the fitted straight line corresponds to the box-dimension value of an object. For details, consult Seidel ([36]).
Statistical analysis
Shapiro-Wilk test and visual assessment (histograms) were used to check for normal distribution of the data. Levene’s test was applied to assess the assumption of variance homogeneity. Significant differences in growth responses, biomass allocation patterns, and structural complexity were tested with Kruskal-Wallis non-parametric test, as the data was not normally distributed, and the variance homogeneity could not be assumed. The differences were tested both between species and across different light levels as well as within species between different light levels and between species across different light levels. Whenever differences were significant, a pairwise Wilcoxon rank-sum test with Bonferroni adjustment was used as a post-hoc test to calculate comparisons between the groups.
On two occasions, for the SLA of P. sylvestris and interspecific comparison of belowground box-dimensions at 100% light availability, the p-values from Kruskal-Wallis test showed significant differences. In contrast, the post-hoc test did not reveal which groups are different from one another. This outcome is most likely due to Bonferroni adjustment since it is known to be one of the most conservative adjustments for family-wise error.
The significance level for all tests was p ≤ 0.05. All statistical analyses and tests were performed in the free software environment R version 3.5.2 (R Development Core Team).
Results
Growth
Relative height and diameter growth were significantly different between species, irrespective of light availability (p<0.001 - Fig. 2). Relative height growth ranged from 60% for P. sylvestris, C. betulus, and A. pseudoplatanus down to 10% for P. abies and A. alba, on average. The relative height increment of P. sylvestris was significantly higher than the relative height increment of the less shade-tolerant conifer species P. abies and A. alba (p<0.001 - Fig. 2). A comparable relationship was not observed between deciduous species or when pooling all tree species.
Fig. 2 - Intra- and interspecific differences in relative height (cm cm-1) and diameter (mm mm-1) growth. Uppercase letters indicate significant interspecific differences between relative height and diameter growth, irrespective of light availability (n=120). Lowercase letters indicate significant intraspecific differences between relative height and diameter growth along the light gradient (n=40). Relative diameter or height growths with the same letter are not significantly different between the species or within species between different light levels. Light availability ranges from 15% - 100%. Species are arranged from the least to the most shade-tolerant, according to Ellenberg & Leuschner ([13]). Species: Ps - P. sylvestris < Qp - Q. petrea < Pa - P. abies < Cb - C. betulus = Ap - A. pseudoplatanus < Aa - A. alba = Fs - F. sylvatica. The y-axis scale of the two graphs is different.
C. betulus and A. pseudoplatanus had the highest relative diameter growth, whereas Q. petrea, P. abies, and A. alba had the lowest one, on average (Fig. 2). No relationship between species’ shade tolerance and higher or lower relative diameter increment was observed.
Relative height growth did not increase with increasing light availability across species and was 35%, on average (Fig. 2). Only for C. betulus, an increase in relative height growth along the light gradient could be detected (p<0.001).
Light availability affected relative diameter growth of F. sylvatica, A. pseudoplatanus, and P. sylvestris seedlings (p<0.05), but only the relative diameter growth of P. sylvestris increased along the light gradient (Fig. 2). In other cases, there were no significant differences between the relative diameter increment at 15% and 100% light availability.
Since there was no pronounced effect of light on the seedling growth, it was not possible to test if the relative height and diameter growth increases more strongly for the light-demanding species.
Biomass production and allocation
There were inter- and intra-specific differences between the total biomass and along the light gradient. An increase in total biomass with increasing light availability could be detected for F. sylvatica, C. betulus, P. abies, and P. sylvestris (p<0.05 - Fig. 3).
Fig. 3 - Total biomass (g) along the light gradient. Letters indicate significant differences of mean total biomass (g) within species between different light levels (n=8). Species are arranged from the least to the most shade-tolerant, according to Ellenberg & Leuschner ([13]). Species: Ps - P. sylvestris < Qp - Q. petrea < Pa - P. abies < Cb - C. betulus = Ap - A. pseudoplatanus < Aa - A. alba = Fs - F. sylvatica.
The total biomass of A. alba, A. pseudoplatanus, and P. abies was on average by 67 grams higher than the total biomass of the other species across all light levels. However, at 100% light availability, the interspecific differences in total biomass became less pronounced, compared to the lower light levels. Even though A. alba, A. pseudoplatanus, and P. abies still had the highest total biomass, it was no longer significantly different from the other species (Fig. 3).
No relationship was detected between species’ shade tolerance rankings and total biomass increase with increasing light availability. With the exception that under 15% and 35% light availability, the total biomass of P. sylvestris was significantly lower than that of P. abies and A. alba (p<0.05).
RMF did not increase with increasing light availability for any of the studied species. Nevertheless, there was a general increase in the RMF of conifers with increasing species’ shade tolerance (Tab. 1). However, the RMF of P. sylvestris was significantly lower than the RMF of P. abies and A. alba only at 35% and 100% light availability (p<0.05). No relationships were observed between the biomass allocation to roots and species’ shade tolerance for deciduous species. Only the RMF of A. pseudoplatanus changed significantly with increasing light availability (p<0.05 - Tab. 1). The RMF of A. pseudoplatanus was the highest at 100% light availability, but it was not significantly different from the RMF at 15% light availability (p>0.05).
Tab. 1 - Mean biomass fractions by species and light levels. Results are presented as mean values of respective plant mass fractions (g g-1) ± standard deviation (SD). Letters indicate significant differences. Biomass fractions of species with the same letter are not significantly different. For intraspecific comparisons, no letters mean no intraspecific differences. Species are arranged from the least to the most shade-tolerant, according to Ellenberg & Leuschner ([13]). Species: Ps - P. sylvestris < Qp - Q. petrea < Pa - P. abies < Cb - C. betulus = Ap - A. pseudoplatanus < Aa - A. alba = Fs - F. sylvatica. (LMF): Needle/leaf mass fraction (g g-1) ± SD; (BMF): branch mass fraction (g g-1) ± SD; (SMF): stem mass fraction (g g-1) ± SD; (RMF): root mass fraction (g g-1) ± SD; (IntraS): intraspecific comparison within species and between light levels; (InterS): interspecific comparison between species and light levels.
Species | Light (%) |
LMF | IntraS LMF | InterS LMF | BMF | IntraS BMF | InterS BMF | SMF | IntraS SMF | InterS SMF | RMF | IntraS RMF | InterS RMF |
---|---|---|---|---|---|---|---|---|---|---|---|---|---|
Ps | 15 | 0.36 ± 0.18 | - | ab | 0.05 ± 0.04 | a | ab | 0.30 ± 0.08 | - | a | 0.29 ± 0.13 | - | ab |
35 | 0.41 ± 0.06 | - | a | 0.06 ± 0.02 | a | a | 0.27 ± 0.04 | - | a | 0.26 ± 0.05 | - | a | |
100 | 0.37 ± 0.06 | - | a | 0.10 ± 0.02 | b | ab | 0.26 ± 0.06 | - | a | 0.26 ± 0.07 | - | a | |
Qp | 15 | 0.11 ± 0.05 | - | a | 0.03 ± 0.01 | a | a | 0.29 ± 0.08 | a | a | 0.57 ± 0.07 | - | c |
35 | 0.13 ± 0.01 | - | b | 0.02 ± 0.01 | b | b | 0.24 ± 0.06 | ab | abc | 0.61 ± 0.06 | - | b | |
100 | 0.13 ± 0.04 | - | bc | 0.03 ± 0.03 | ab | cd | 0.19 ± 0.03 | b | ab | 0.64 ± 0.08 | - | b | |
Pa | 15 | 0.21 ± 0.09 | - | ab | 0.16 ± 0.03 | - | c | 0.25 ± 0.06 | - | a | 0.37 ± 0.06 | - | a |
35 | 0.25 ± 0.02 | - | c | 0.14 ± 0.02 | - | c | 0.19 ± 0.03 | - | bd | 0.42 ± 0.05 | - | c | |
100 | 0.23 ± 0.05 | - | d | 0.14 ± 0.02 | - | e | 0.19 ± 0.04 | - | ab | 0.44 ± 0.04 | - | c | |
Cb | 15 | 0.18 ± 0.05 | - | ab | 0.09 ± 0.03 | - | b | 0.28 ± 0.06 | - | d | 0.45 ± 0.07 | - | abc |
35 | 0.19 ± 0.02 | - | d | 0.06 ± 0.01 | - | a | 0.31 ± 0.07 | - | ac | 0.44 ± 0.08 | - | cd | |
100 | 0.18 ± 0.03 | - | bd | 0.07 ± 0.02 | - | ac | 0.26 ± 0.06 | - | a | 0.48 ± 0.08 | - | c | |
Ap | 15 | 0.18 ± 0.06 | - | ab | 0.02 ± 0.02 | - | a | 0.35 ± 0.09 | a | a | 0.46 ± 0.14 | ab | abc |
35 | 0.21 ± 0.04 | - | cd | 0.01 ± 0.01 | - | b | 0.35 ± 0.05 | a | c | 0.43 ± 0.06 | a | cd | |
100 | 0.18 ± 0.03 | - | bd | 0.01 ± 0.01 | - | a | 0.22 ± 0.09 | b | ab | 0.58 ± 0.09 | b | bc | |
Aa | 15 | 0.23 ± 0.02 | - | b | 0.16 ± 0.02 | - | c | 0.15 ± 0.02 | - | b | 0.46 ± 0.03 | - | bc |
35 | 0.24 ± 0.02 | - | c | 0.14 ± 0.02 | - | c | 0.14 ± 0.03 | - | d | 0.48 ± 0.04 | - | cd | |
100 | 0.23 ± 0.13 | - | abcd | 0.14 ± 0.03 | - | be | 0.13 ± 0.04 | - | b | 0.50 ± 0.10 | - | bc | |
Fs | 15 | 0.12 ± 0.02 | - | a | 0.10 ± 0.02 | - | b | 0.27 ± 0.03 | - | a | 0.51 ± 0.03 | - | bc |
35 | 0.14 ± 0.02 | - | b | 0.08 ± 0.02 | - | a | 0.25 ± 0.04 | - | ab | 0.53 ± 0.05 | - | bd | |
100 | 0.12 ± 0.03 | - | c | 0.09 ± 0.02 | - | ab | 0.26 ± 0.09 | - | a | 0.53 ± 0.11 | - | bc |
None of the species allocated more biomass towards leaves and needles as a response to decreased light availability (Tab. 1). Light-demanding P. sylvestris had the highest LMF along the light gradient. However, it was significantly higher only at 35% light availability (p<0.05). Q. petrea and F. sylvatica, the most light-demanding and the most shade-tolerant of the studied deciduous species, had significantly lower LMF than A. pseudoplatanus and C. betulus. LMF was not related to species shade tolerance and was not different between coniferous and deciduous species.
For most species, biomass allocation to branches remained relatively constant along the light gradient. P. sylvestris and Q. petrea - two of the most light-demanding of the studied species - reacted to increasing light availability by focusing the biomass allocation to branches (p<0.05 - Tab. 1). Even though the BMF of P. sylvestris doubled from 15% to 100% light availability, it was still the lowest among conifers (p<0.05 under 15% and 35% light availability). The changes in BMF of Q. petrea, though statistically significant, were trivial. The stem mass fraction (SMF) of Q. petrea decreased by 35 %, on average, with increasing light availability. The SMF of A. pseudoplatanus also decreased with increasing light availability (p<0.05). Other species, except C. betulus, showed a similar trend, but the reduction was not significant.
Leaf and plant morphology
SLA of all studied species decreased with increasing light availability (p<0.05 - Fig. 4a, Fig. 4b). However, for P. sylvestris, it was only possible to detect differences from the Kruskal-Wallis test, but not the post-hoc test (for explanation see the Materials and Methods section).
Fig. 4 - Specific leaf area (SLA, cm2 g-1) of the studied conifer (a) and deciduous (b) tree species. Letters indicate significant differences of SLA within species between different light levels (n=8). SLA of species with the same letter is not significantly different between the light levels. Species are arranged from the least to the most shade-tolerant, according to Ellenberg & Leuschner ([13]). Species: Ps - P. sylvestris < Qp - Q. petrea < Pa - P. abies < Cb - C. betulus = Ap - A. pseudoplatanus < Aa - A. alba = Fs - F. sylvatica. The y-axis scale of the two graphs is different.
Deciduous trees had significantly higher SLA than coniferous trees (p<0.001 - Fig. 4a, Fig. 4b). The least shade-tolerant conifer species P. sylvestris had the lowest SLA along the light gradient (Fig. 4a). SLA of the most shade-tolerant deciduous species F. sylvatica was higher than the SLA of other deciduous species along the light gradient but did not differ significantly from Q. petrea under 15% light availability and C. betulus under 35% and 100% light availability. Hence, the relationship between higher species’ shade tolerance and higher SLA for deciduous species was inconclusive.
F. sylvatica was the only species that increased the aboveground box-dimension (p<0.05), i.e., the morphological complexity along the light gradient (Tab. 2). Though a similar trend could be observed for P. abies, Q. petrea and P. sylvestris, the increase was not significant for these species.
Tab. 2 - Above- and below-ground box-dimensions (Db) by species and light levels. Results are presented as the mean above and belowground box-dimension values ± standard deviation (SD). Letters indicate significant differences. Box dimensions of species with the same letter are not significantly different. Species are arranged from the least to the most shade-tolerant, according to Ellenberg & Leuschner ([13]). Species: Ps - P. sylvestris < Qp - Q. petrea < Pa - P. abies < Cb - C. betulus = Ap - A. pseudoplatanus < Aa - A. alba = Fs - F. sylvatica. (AG Db): aboveground box-dimension ± SD; (BG Db): aboveground box-dimension ± SD; (IntraS): intraspecific comparison within species and between light levels - no letters at intraspecific comparisons mean that there were no significant differences; (InterS): interspecific comparison between species and light levels.
Species | Light (%) |
AG Db | IntraS | InterS | BG Db | IntraS | InterS |
---|---|---|---|---|---|---|---|
Ps | 15 | 1.3 ± 0.3 | - | ab | 1.2 ± 0.2 | a | a |
35 | 1.5 ± 0.3 | - | ab | 1.4 ± 0.1 | ab | a | |
100 | 1.6 ± 0.2 | - | abc | 1.5 ± 0.1 | b | - | |
Qp | 15 | 1.0 ± 0.0 | - | a | 1.4 ± 0.1 | - | ab |
35 | 1.1 ± 0.1 | - | c | 1.4 ± 0.2 | - | ab | |
100 | 1.1 ± 0.1 | - | ab | 1.4 ± 0.2 | - | - | |
Pa | 15 | 1.6 ± 0.2 | - | b | 1.6 ± 0.1 | - | c |
35 | 1.7 ± 0.2 | - | a | 1.6 ± 0.1 | - | bc | |
100 | 1.7 ± 0.2 | - | c | 1.6 ± 0.1 | - | - | |
Cb | 15 | 1.1 ± 0.1 | - | a | 1.4 ± 0.1 | a | ab |
35 | 1.1 ± 0.1 | - | c | 1.6 ± 0.1 | b | bc | |
100 | 1.1 ± 0.1 | - | a | 1.6 ± 0.1 | ab | - | |
Ap | 15 | 1.1 ± 0.1 | - | a | 1.6 ± 0.2 | - | bc |
35 | 1.1 ± 0.1 | - | c | 1.6 ± 0.1 | - | abc | |
100 | 1.1 ± 0.1 | - | a | 1.7 ± 0.2 | - | - | |
Aa | 15 | 1.6 ± 0.3 | - | b | 1.8 ± 0.2 | - | c |
35 | 1.6 ± 0.2 | - | a | 1.8 ± 0.1 | - | c | |
100 | 1.6 ± 0.2 | - | bc | 1.7 ± 0.1 | - | - | |
Fs | 15 | 1.1 ± 0.1 | a | a | 1.6 ± 0.1 | - | abc |
35 | 1.2 ± 0.1 | ab | bc | 1.6 ± 0.2 | - | abc | |
100 | 1.3 ± 0.2 | b | abc | 1.7 ± 0.2 | - | - |
The belowground box-dimensions of C. betulus and P. sylvestris seedlings were positively related to increasing light availability (p<0.05). Belowground box-dimensions of P. sylvestris increased consistently along the light gradient. Whereas for C. betulus, the differences were significant only between 15% and 35% light availability (p<0.05).
Coniferous species had higher aboveground box-dimensions (1.6 ± 0.3) than the deciduous species (1.1 ± 0.1; p>0.05 - Tab. 2). However, there were no significant differences within the coniferous and deciduous species groups (p>0.05). Respectively, no relationship between species’ shade tolerance and aboveground morphological complexity was found.
The belowground box-dimension of light-demanding P. sylvestris was significantly lower than of the more shade-tolerant conifers (p<0.001; A. alba = P. abies > P. sylvestris). A similar relationship was not observed for deciduous species.
Discussion
Height and diameter growth
We found differences in relative height and diameter growth between species (H 1.1), but no pronounced relative height and diameter growth increase with increasing light availability (H 1.2). Consequently, we could not support our hypothesis 1.3 - that relative height and diameter growth increase with increasing light availability and that the increase is stronger for the light-demanding species.
Although shade-tolerant and light-demanding species employ different growth strategies when it comes to limited light conditions, species are known to generally respond to increased light availability by pronounced vertical and lateral growth ([3]). The lack of a positive effect of light on growth was not anticipated, and is contrary to the vast majority of scientific findings ([8], [21]). This result may be caused by the relatively short time in which the seedlings were exposed to different light conditions. More specifically, we argue that because trees were grown under open field conditions, both in the nursery and for the first year of the experiment, a delayed response to limited light availability is likely ([47], [39]). The short exposure time might have affected both relative height and diameter growth along the light gradient and the species-specific growth rates. Hence, we expect to see intra- and inter-specific differences that are more pronounced at the later stages of the experiment.
Biomass production and allocation
Total biomass increased significantly with increasing light availability for F. sylvatica, C. betulus, P. abies, and P. sylvestris. However, we did not find that the increase was more pronounced for light-demanding species. Hence, we can only partly confirm hypothesis 2.1 that the total biomass increases with increasing light availability. The positive effect of light availability on total biomass production has been nearly indisputable ([24], [7]). However, when studying the growth of young seedlings, the initial size of the seedling might have a more pronounced effect on growth than light availability ([44]).
According to the balanced growth hypothesis ([40]), we hypothesized that RMF increases, and LMF decreases with increasing light availability (H 2.2). However, neither of the species changed the LMF along the light gradient, and only A. pseudoplatanus slightly increased the RMF. Hence, we could not support the hypothesis. P. sylvestris, the least shade-tolerant species, increased the branch mass fraction with increasing light availability. We did not find it to be a general trend amongst less shade-tolerant species. Consequently, we could confirm that BMF remains constant for shade-tolerant species, but not that it increases with increasing light availability for all light-demanding species (H 2.3).
Similarly, to the relative height and diameter growth, our results here contradict the common expectations. Even though the balanced growth hypothesis has been widely confirmed ([33], [34]), there have also been some studies that did not detect the expected allocation patterns for young seedlings as a function of light or a distinctly improved species performance with increasing light availability ([12]). Additionally, it is also known that plasticity negatively correlates with tree size ([23]) and that trees are generally expected to have more pronounced differences in shade-tolerance in the seedling stage ([10]). However, some authors have found that young seedlings are well adapted to low light conditions, regardless of the presumed shade tolerance ([25], [47]).
Therefore, we argue that the main reason for this is a high adaptive potential of seedlings to low light conditions and the short time since plants were exposed to limited light. Combined with the high vigor of the seedlings grown in nurseries under favorable conditions and full light, this has most likely facilitated the delayed response.
Leaf and plant morphology
We found that SLA increased with increasing light availability, which confirms our hypothesis 3.1 and is in line with previous studies ([29], [26]). Leaves are the most plastic plant organ ([28]) and, thus, the first to show adaptations to the changing light environment. Therefore, when light availability is low, trees increase their photosynthetic surface area by forming lighter or larger leaves, or both, thus increasing the SLA ([16], [39]).
Shade tolerant species growing in temperate forests tend to have generally higher SLA than less shade-tolerant species ([12], [39]). Although our findings were inconclusive, we did observe that A. alba and F. sylvatica, the most shade-tolerant species, had the highest SLA, among the coniferous and deciduous species. However, the SLA did not yet continue to gradually decrease with decreasing species’ shade tolerance rankings. Therefore, we can only partly confirm our hypothesis that the shade-tolerant species have a higher SLA (H 3.2). Higher SLA of shade-tolerant species contributes to the light interception at a lower cost via the production of leaf area, while less shade-tolerant species prioritize investing in more durable leaves (higher leaf life-span) and thus have lower SLA ([18]). The differences can be partly explained by higher phenotypic plasticity (e.g., leaf size) of shade-tolerant species and higher physiological plasticity (e.g., maximum photosynthetic rate) of light-demanding species ([42]).
In our study, conifer seedlings had higher aboveground box-dimensions on average (Db = 1.6) compared to the seedlings of deciduous tree species (Db = 1.1 - Tab. 2). The box-dimension values can theoretically range from one to three, and values close to one indicate that the deciduous trees were nearly branch-less and rather pole-like ([38]). However, the BMF of C. betulus and F. sylvatica ranged from 6-10%, on average, but still had box-dimensions of only 1.1. The low box-dimension values are because, overall, there were hardly any branches, and they were small and with very few bifurcations. Higher box-dimensions of the coniferous species are most likely a result of branch whorls, characteristic to all of the studied conifers, and have been reported before ([36]). Having whorls resulted in more developed crowns with multiple orders of branches, as opposed to all the deciduous species, except F. sylvatica. In the upcoming years, we expect that also the deciduous trees will form more complex structures via increased branching.
Only the aboveground structural complexity of F. sylvatica increased along the light gradient. This finding is in line with previous studies which found F. sylvatica to have higher morphological plasticity than less shade-tolerant species ([41], [34]). Surprisingly, no other species reacted to increased light availability yet, and our hypothesis that structural complexity of plant architecture increases with increasing light availability (H 3.3) could only be supported for F. sylvatica, but not for the other species. Trees are expected to promote branch growth in order to optimize light interception ([15]) and not to lose contact with the light ([1]). However, when the light availability is not limited, trees are to develop closer to their genetically predefined structure ([37]). Hence, differences in the structural complexity of plant architecture, mainly in terms of branch formation of plants, are expected along the light gradient.
P. sylvestris and C. betulus were the only species to show increased belowground structural complexity with increasing light. However, we are aware of several limitations when using box-dimensions (calculated from 3D point clouds) for belowground structural complexity assessment. Firstly, when assessing root structural complexity using a destructive harvest, we are not able to assess the actual root distribution in soil. Secondly, the laser beams (Ø 3 mm) might not hit the finer roots or refract if the roots are not completely dry, thus leading to possible underestimations. Therefore, we would interpret these findings with caution.
Since there was a limited response in structural complexity of plant architecture along the light gradient after one year of exposure to different light conditions, we also could not confirm that the structural complexity of light-demanding species increases more with increasing light availability (H 3.4). Such an outcome was expected because of the more pronounced effect of light limitation and hence, a stronger response of light-demanding species to reduced light stress.
Similarly, as with growth and biomass allocation patterns, we argue that the short time (one vegetation period) spent under shaded conditions is the main reason for the delayed response in intraspecific differences of structural complexity of plant architecture. Additionally, we expect that trees will develop more complex crowns, and the differences will become more pronounced with ontogeny ([47], [17]).
Conclusions
In our study, we could not generally observe the expected effect of light availability on growth rates, biomass allocation patterns, and structural complexity of plant architecture or see pronounced differences between tree species with varying shade tolerance. Nevertheless, we found the initial adaptation all species made by increasing SLA with decreasing light availability. Assuming the potentially delayed reaction of less plastic plant organs, we have a reason to assume that in the following stages of the experiment, we will see increasingly pronounced effects in the morphological adaptations of the tree species. We also expect that the intra- and interspecific differences will become stronger with time.
Acknowledgements
We thank the technicians of the Department of Silviculture and Forest Ecology of the Temperate Zones, University of Göttingen, Germany for assistance with sampling and data collection and student assistants for scanning the seedlings and processing the point clouds. In addition, we thank the anonymous reviewers of the manuscript for recommendations and constructive comments.
Funding
This work was supported by The German Research Foundation (DFG) through Grant SE 2383/5-1 provided to Dominik Seidel.
References
Online | Gscholar
Gscholar
Gscholar
Gscholar
Gscholar
CrossRef | Gscholar
Authors’ Info
Authors’ Affiliation
Christian Ammer 0000-0002-4235-0135
Dominik Seidel 0000-0003-4131-9424
Department of Silviculture and Forest Ecology of the Temperate Zones, Faculty of Forest Sciences and Forest Ecology, Georg-August-University of Göttingen, Büsgenweg 1, 37077 Göttingen (Germany)
Forest and Agroforest Systems, Technical University of Munich (TUM), Hans-Carl-von-Carlowitz-Platz 2, 85354 Freising (Germany)
Corresponding author
Paper Info
Citation
Bebre I, Annighöfer P, Ammer C, Seidel D (2020). Growth, morphology, and biomass allocation of recently planted seedlings of seven European tree species along a light gradient. iForest 13: 261-269. - doi: 10.3832/ifor3370-013
Academic Editor
Claudia Cocozza
Paper history
Received: Feb 07, 2020
Accepted: May 05, 2020
First online: Jul 03, 2020
Publication Date: Aug 31, 2020
Publication Time: 1.97 months
Copyright Information
© SISEF - The Italian Society of Silviculture and Forest Ecology 2020
Open Access
This article is distributed under the terms of the Creative Commons Attribution-Non Commercial 4.0 International (https://creativecommons.org/licenses/by-nc/4.0/), which permits unrestricted use, distribution, and reproduction in any medium, provided you give appropriate credit to the original author(s) and the source, provide a link to the Creative Commons license, and indicate if changes were made.
Web Metrics
Breakdown by View Type
Article Usage
Total Article Views: 38501
(from publication date up to now)
Breakdown by View Type
HTML Page Views: 32933
Abstract Page Views: 2634
PDF Downloads: 2274
Citation/Reference Downloads: 2
XML Downloads: 658
Web Metrics
Days since publication: 1835
Overall contacts: 38501
Avg. contacts per week: 146.87
Article Citations
Article citations are based on data periodically collected from the Clarivate Web of Science web site
(last update: Mar 2025)
Total number of cites (since 2020): 5
Average cites per year: 0.83
Publication Metrics
by Dimensions ©
Articles citing this article
List of the papers citing this article based on CrossRef Cited-by.
Related Contents
iForest Similar Articles
Research Articles
Growth and physiological acclimation to shade in young plants of Adesmia bijuga Phil., a critically endangered species in central Chile
vol. 14, pp. 307-312 (online: 01 July 2021)
Research Articles
Conservation of Betula oycoviensis, an endangered rare taxon, using vegetative propagation methods
vol. 13, pp. 107-113 (online: 23 March 2020)
Short Communications
Effect of four levels of shade on survival, morphology and chlorophyll fluorescence of Nothofagus alessandrii container-grown seedlings
vol. 8, pp. 638-641 (online: 08 January 2015)
Research Articles
Leaf morphology of progenies in Q. suber, Q. ilex, and their hybrids using multivariate and geometric morphometric analysis
vol. 11, pp. 90-98 (online: 31 January 2018)
Research Articles
Gas exchange, biomass allocation and water-use efficiency in response to elevated CO2 and drought in andiroba (Carapa surinamensis, Meliaceae)
vol. 12, pp. 61-68 (online: 24 January 2019)
Research Articles
Substrates and nutrient addition rates affect morphology and physiology of Pinus leiophylla seedlings in the nursery stage
vol. 10, pp. 115-120 (online: 02 October 2016)
Research Articles
Chitosan oligosaccharide addition affects current-year shoot of post-transplant Buddhist pine (Podocarpus macrophyllus) seedlings under contrasting photoperiods
vol. 10, pp. 715-721 (online: 27 July 2017)
Research Articles
Use of alternative containers for promoting deep rooting of native forest species used for dryland restoration: the case of Acacia caven
vol. 10, pp. 776-782 (online: 02 September 2017)
Research Articles
Use of overburden waste for London plane (Platanus × acerifolia) growth: the role of plant growth promoting microbial consortia
vol. 10, pp. 692-699 (online: 17 July 2017)
Research Articles
First vs. second rotation of a poplar short rotation coppice: leaf area development, light interception and radiation use efficiency
vol. 8, pp. 565-573 (online: 27 April 2015)
iForest Database Search
Search By Author
Search By Keyword
Google Scholar Search
Citing Articles
Search By Author
Search By Keywords
PubMed Search
Search By Author
Search By Keyword