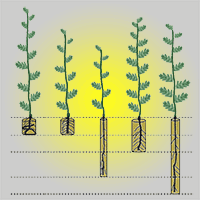
Use of alternative containers for promoting deep rooting of native forest species used for dryland restoration: the case of Acacia caven
iForest - Biogeosciences and Forestry, Volume 10, Issue 5, Pages 776-782 (2017)
doi: https://doi.org/10.3832/ifor2101-010
Published: Sep 02, 2017 - Copyright © 2017 SISEF
Research Articles
Abstract
The size of a container determines the development and quality of root systems. In the case of taprooted forest species used for dryland reforestation, deeper containers may favour early root development and, consequently, better soil profile colonization after outplanting. Although research on container design for worldwide tree species has been developed in the last decades, technical solutions for containerized forest species with a taproot system have been poorly documented. We present a case study using Acacia caven (Mol.) Mol., which has fast-growing taproots and long lateral and superficial roots. The aim of this study is to evaluate the effects of different containers on rooting volume in the early morphological development of A. caven seedlings. Ten day-old seedlings were cultivated in five different PVC container types varying in volume, width and length (T440-Short, T440-Long, T880-Short, T880-Long, and T440-C), in a completely randomized design for one growing season. At the end of the study, whole seedling samples were destroyed to assess taproot length, lateral root biomass, and total root/shoot dry biomass. To evaluate the potential plant capacity for developing new roots, a subsequent experiment using the root growth potential test was performed successfully. Results showed that change in root volume distribution (short vs. elongated containers) had the greatest influence on seedling quality, whereas the size of container (small volume vs. large) was of minor importance. Elongated containers (35 cm to 40 cm in length) with self-pruning basal roots produced seedlings with smaller shoot/root ratios, longer root systems, and a greater ability to restart new root growth in deeper container strata. Elongated containers also prevented taproot deformation. The present study suggests that it would be appropriate to rethink container design for seedlings of deep-rooted xerophytic species destined for water-limited transplanting conditions.
Keywords
Native Tree Domestication, Root Growth Potential, Root Morphology, Seedling Quality
Introduction
Acacia trees (Leguminosae) are commonly used around the world in the restoration of degraded forest ecosystems ([14], [20], [19]). Among these, Acacia caven is one of the most widespread Neotropical South American tree species (latitude 18° to 37° S - [4]). It has been commonly used for the reforestation of degraded arid regions ([13]), as its massive and deep-rooted system allows it to cope with a sudden abundance of water ([4]). This species generates positive interactions (facilitation) with co-occurring tree species that have lower water-stress resistance ([3]), as it improves both the soil nutritional level due to its high nitrogen fixation potential and the microclimatic conditions under the canopy ([4]).
Deep roots, or taproots, defined as roots that grow to depths greater than 1 m ([24]), are functionally important for drought-adapted woody species ([40], [9], [29]). Their main functions are hydraulic redistribution, resistance to embolism and to physical-chemical weathering, and C sequestration in deep soil strata, among others ([6], [21]). As for early seedling survival and establishment, taproots allow rapid deep growth toward deep water sources available in soils, particularly before the first dry season ([7], [31]). Indeed, a number of studies have demonstrated a positive relationship between root length and early seedling survival in arid ([22]), semiarid ([29]), and tropical dry forest ecosystems ([27]).
From a silvicultural point of view, a main challenge for nurseries is to produce containerized seedlings that mimic the root size and architecture of naturally generated seedlings. However, in most cases, containers used for nursery propagation result in seedlings with restricted root development and a completely different root architecture compared to natural conditions ([47], [15]). This is the case with small containers (< 400 mL), as broadly described in the literature ([39], [11], [37], [1], [49]). Chilean nurseries commonly use small containers such as black polyethylene bags, which are quite shallow with typical volumes lower than 400 mL ([29]). In addition, black polyethylene bags absorb solar radiation and increase substrate temperatures, which retards root growth at temperatures greater than 30 °C. Reduced volume in containers does not promote lateral root self-pruning at early growth stages, and this often results in root deformation, which further restricts growth ([12]). Limitations of small containers result in serious morphological constraints and higher shoot/root ratios. This has a strong and negative impact on seedlings’ water economy after they are outplanted, particularly under arid and semiarid climate conditions ([39], [46]).
In the last decade, there has been an increasing interest in improving seedling quality of deep-rooting Mediterranean tree species, including the redesign of propagation containers ([8], [25], [30]). Traditional nursery cultural practices meant to improve the quality of seedlings have been developed mostly for tree species belonging to North American boreal forests ([18], [17]), which tend to develop shallow root systems ([41]). By contrast, the plant quality in xeric environments is related to the development of drought-tolerant traits ([10]) such as low specific leaf area, large stem diameter, low shoot/ root ratio, and large root volume ([44]). These traits need to be achieved at early propagation stages. In this context, large-volume containers with better volume distribution would facilitate taproot growth and improve whole plant quality for xerophytic species, assuring proper root functionality for the seedlings’ survival and establishment in soils with low water availability ([5]). Several studies have reported on a positive relationship between large-volume containers, root systems ([25]) and seedlings’ total height ([2]). An increase of up to 40% in root biomass has been obtained by doubling container volume ([33]), and significant improvements in root growth capacity, root hydraulic conductance, and stomatal conductance have been obtained with containers above 500 mL in volume and 25 cm in depth ([8]). Furthermore, spiral root prevention, generation of lateral roots, and improvements in root distribution and fibrosity have resulted from the inclusion of container walls to promote self-pruning of basal and lateral roots ([37]).
No documented studies provide evidence concerning the quality of plant attributes of A. caven seedling production, which are commonly used in the reforestation of highly degraded dry land. It is necessary to evaluate the application of nursery practices commonly used for other productive tree species ([36]) to improve nursery management of deep-rooted xerophytic species. In the present study, we evaluate the effect of container size and volume distribution on A. caven seedlings during early plant development.
Materials and methods
Experimental conditions and plant material
Acacia caven seedlings were cultivated in a growth room at Centro de Investigación Minera y Metalúrgica (CIMM) in Santiago, Chile (33° 26′ S - 74° 40′ W). Inside the controlled growth room, the established mean temperature was 23 ± 2 °C, relative humidity was 46%, and light intensity was 273 µmol s-1 m-2, with a 12/12 h photoperiod. Seedlings were produced from seeds collected from individual trees growing in Elqui Province, north-central Chile (29° 49′ S - 70° 48′ W). The seeds were subjected to chemical scarification with concentrated sulfuric acid (technical quality) for 120 minutes before they were used. They were then germinated in 1-L containers filled with A-6 perlite substrate (Harborlite®). The average final seedling size (length) before transplantation to experimental containers was 3 cm for shoots, 5 cm for root systems, and a pair of well-developed true leaves. No root malformation at the taproot was observed. Seedlings were individually transplanted to experimental containers filled with substrate (described below - Fig. 1). Water content of the containers was daily checked and when it reached 40% field capacity, demineralized water was added up to 80% field capacity. Seedlings were fertilized twice along the assay with 40 mL of a modified Hoagland’s solution containing 150 mg N L-1, 80 mg P L-1, and 100 mg K L-1. Seedlings were grown for six months until control seedlings reached 60 cm in height, which is the average size equivalent to a plant of this species after one nursery season in central Chile.
Fig. 1 - Graphical representation of container types (treatments) used for Acacia caven seedlings’ growth under experimental conditions. Treatments (container volume, container length): T440-C (440 mL, 10 cm); T440-Short (440 mL, 10 cm); T440-Long (440 mL, 35 cm); T880-Short (880 mL, 20 cm); T880-Long (880 mL, 45 cm).
Experimental design and treatments
The study was conducted in two stages. The first stage consisted of an experiment with five treatments distributed randomly over the benches in the plant growth room, using containers of different sizes and with varying volume distributions: T440-Short (440 mL, 10 cm in length, 7.5 cm in diameter), T440-Long (440 mL, 35 cm in length, 4.0 cm in diameter), T880-Short (880 mL, 20 cm in length, 7.5 cm in diameter), T880-Long (880 mL, 45 cm in length, 5.0 cm in diameter), plus the control treatment T440-C (440 mL, 10 cm in length, 7.5 cm in diameter - Fig. 1). To obtain different sizes and rooting volume distributions, containers with the same volume (440 mL or 880 mL) but different lengths (from 10 cm to 45 cm in length) were used (Fig. 1). Experimental containers were made of PVC (polyvinyl chloride) tubes. The control treatment was a 12 × 15 cm black polyethylene bag of 440 mL, which is the most commonly used container in local tree nurseries ([29]). To promote self-pruning of basal roots and good drainage, all PVC containers were cut vertically in their bases (3 cm long every 3 cm), and a 2 × 2 mm plastic mesh opening was glued to the base. To favour lateral self-pruning of secondary roots, side slots were made along each PVC container. PVC containers were left suspended (in contact with air) on a plastic structure. Seedling density was 82 plants per m2. The distribution of treatments in the plastic structure was randomized with 16 replications per treatment (80 seedlings in total).
The substrate used to fill the experimental containers up to 88% of their volume was a mixture of one part A-6 perlite (Harborlite®), one-part peat (Kekkilä DSM), and two parts loam soil. To maintain constant substrate density in all treatments, the correlation between weight of the substrate and container volume was considered. The substrate volume used for the treatments T440-C, T440-Short, and T440-Long was 385 mL, while a volume of 770 mL was used for the treatments T880-Short and T880-Long.
Seedlings’ morphological measurements
From each treatment, six randomly chosen seedlings were assessed for morphology measurements ([45]). Morphological variables measured were shoot height, root collar diameter (RCD), shoot dry biomass, root dry biomass, shoot height/RCD ratio, and shoot/root dry biomass ratio. Shoot height was measured with a graduated metal ruler from the substrate surface to the apex of the main stem. RCD was measured with a digital calliper below the insertion of the cotyledons. To determine dry biomass, plant tissues were washed with tap water and dried at 45 °C in an air-forced oven until constant weight was reached. Taproot length was measured with scanned images obtained from the root system, after being thoroughly washed with tap water. For taproot length, container depth and the presence of spiral roots were considered. Shoot height and RCD were evaluated once a month for all plants. The remaining variables were evaluated only at the end of the assay, six months after establishment.
Root growth potential tests
The second stage of the experiment was performed to assess the potential capability of roots to produce new growth after growing in different containers. Therefore, the root growth potential (RGP) test was performed following the methodology used by Ritchie ([38]) and Trubat et al. ([45]). Six plants per treatment were randomly selected at the end of the first stage of experimentation and transplanted into a larger PVC container (70 cm wide). PVC tubes were longitudinally cut into two parts to facilitate the final evaluation. The size of containers varied according to the initial treatment, but the plants had equal space left for further growth (3 cm to each side of the original root plug and 20 cm to the base). The experimental substrate used to fill the containers was A-6 perlite (Harborlite®). The containers were irrigated twice a week with demineralized water up to 100% field capacity. RGP tests lasted for 28 days ([38]), as assessed from a pre-test with the objective of determining the number of days required for A. caven roots to grow in the container without reaching the bottom. After cultivation, each container was longitudinally opened and divided into transverse 10-cm-deep sections; the number of roots larger than 1 cm ([8]) per section was then determined. New root growth in terms of dry biomass was also analysed; new roots were cut by hand, washed, and dried at 45 °C in an air-forced oven until constant weight was reached.
Data analysis
One-way analysis of variance (ANOVA) was used to specify significant differences (P<0.05) among treatments for each response variable evaluated in the first stage of the experiment. For the RGP test, one-way ANOVA was used to determine significant differences (P<0.05) among treatments for each substrate depth section of the containers. Fisher’s LSD test was used to discriminate between treatments (P <0.05). Prior to the ANOVA, data normality was verified by the Shapiro-Wilks test and the homogeneity of variances by Levene’s test. If requirements were not met, data was transformed or analysed using the nonparametric Kruskal-Wallis test. All statistical analyses were performed with InfoStat® software v. 2010.
Results
Morphological parameters
The different container types resulted in significant effects (P<0.05) on A. caven seedlings’ shoot height, RCD, shoot height/ RCD ratio, and taproot length (Tab. 1). In general, containers with higher volume (T880-Short and T880-Long) did not exhibit a clear pattern for morphological parameters when they were compared to containers with lower volume (T440-Short, T440-Long and T440-C - Fig. 1). Seedlings that were grown in 440-mL containers had significantly higher shoot height and shoot height/RCD ratio (P<0.05) for the control treatment compared to the short and elongated containers. RCD was significantly higher (P<0.05) for seedlings grown in short containers (T440-C, T440-Short, and T880-Short), independent of container volume. No significant differences were found between type of container for shoot biomass, root biomass, shoot/root dry biomass ratio, or Dickson quality index (DQI - Tab. 1). Treatment with the largest container (T880-Long) promoted significantly longer taproot length (P <0.05) in relation to the other treatments and control. By contrast, short containers (T440-C, T440-Short, and T880-Short) showed small taproot growth (Tab. 1) and root deformation at the container base. Therefore, container depth influenced taproot length. In addition, the taproot in T440-C was three times longer than that in T440-Short. In T440-C, 67% of the seedlings had spiral roots, while those grown in containers with side holes (T440-Short, T440-Long, T880-Short, and T880-Long) did not have spiral roots (Tab. 1).
Tab. 1 - Morphological parameters of Acacia caven seedlings after six months growing in experimental containers. Mean ± standard error values are given. Different letters indicate significant differences among treatments according to one-way ANOVA and Fisher’s LSD tests (P<0.05). Treatments (container volume, container length): T440-C (440 mL, 10 cm); T440-Short (440 mL, 10 cm); T440-Long (440 mL, 35 cm); T880-Short (880 mL, 20 cm); T880-Long (880 mL, 45 cm).
Morphological parameter |
Treatments | F-value | P-value | ||||
---|---|---|---|---|---|---|---|
T440-C | T440-Short | T440-Long | T880-Short | T880-Long | |||
Shoot height (cm) | 63.4 ± 3.7 a | 50.0 ± 3.8 bc | 41.0 ± 2.6 c | 55.5 ± 4.4 ab | 52.7 ± 3.4 b | 5.13 | <0.05 |
RCD (mm) | 4.0 ± 0.1 a | 3.9 ± 0.2 a | 3.3 ± 0.1 b | 4.0 ± 0.1 a | 3.6 ± 0.1 b | 7.10 | <0.05 |
Shoot height/RCD ratio | 157.6 ± 8.1 a | 126.2 ± 7.4 b | 125.7 ± 6.8 b | 139.6 ± 8.9 ab | 147.9 ± 8.2 ab | 3.06 | <0.05 |
Shoot biomass (g) | 1.6 ± 0.3 | 1.5 ± 0.2 | 0.8 ± 0.1 | 1.9 ± 0.4 | 1.6 ± 0.2 | 2.44 | 0.073 |
Root biomass (g) | 1.1 ± 0.1 | 1.2 ± 0.3 | 0.7 ± 0.1 | 1.4 ± 0.2 | 1.3 ± 0.2 | 1.80 | 0.161 |
Shoot/root ratio | 1.4 ± 0.2 | 1.3 ± 0.2 | 1.1 ± 0.1 | 1.4 ± 0.1 | 1.2 ± 0.1 | 1.01 | 0.422 |
Taproot length (cm) | 18.8 ± 3.5 b | 6.9 ± 0.3 c | 26.1 ± 0.9 ab | 12.6 ± 0.5 bc | 33.7 ± 0.5 a | 24.20 | <0.05 |
Dickson Quality Index (DQI) | 0.18 ± 0.02 | 0.19 ± 0.04 | 0.12 ± 0.01 | 0.20 ± 0.03 | 0.18 ± 0.02 | 1.50 | 0.231 |
Root growth potential test
No significant differences concerning root biomass or total number of new roots were found among treatments for A. caven seedlings after the RGP test (Tab. 2). However, significant effects (P<0.05) were found in terms of root length/container length (%), as expected. Seedlings of the T880-Long treatment tended to produce greater root biomass than the other treatments, including the control. By contrast, the T440-Long treatment tended to produce the lowest root biomass. Comparing short containers of different sizes, we observed that root biomass of T880-Short was 25% larger than that of the T440-Short treatment, because the former has twice the volume. A similar tendency was detected for elongated containers where T880-Long produced twice the biomass of the T440-Long treatment. In terms of the number of new roots, larger containers (T880-Short and T880-Long) tended to produce larger amounts compared to small containers and the control.
Tab. 2 - Root morphology characteristics of Acacia caven seedlings after the root growth potential (RGP) tests. Mean ± standard error values are given. Different letters indicate significant differences among treatments according to one-way ANOVA and Fisher’s LSD tests (P<0.05). Treatments: T440-C (440 mL, 10 cm in length); T440-Short (440 mL, 10 cm in length); T440-Long (440 mL, 35 cm in length); T880-Short (880 mL, 20 cm in length); T880-Long (880 mL, 45 cm in length).
Parameter | Treatments | F-value | P-value | ||||
---|---|---|---|---|---|---|---|
T440-C | T440-Short | T440-Long | T880-Short | T880-Long | |||
Root biomass (mg) | 115.9 ± 29.7 | 87.8 ± 11.8 | 77.0 ± 12.2 | 109.9 ± 28.1 | 147.1 ± 31.3 | 1.26 | 0.311 |
Root number | 71 ± 15 | 71 ± 5 | 60 ± 9 | 94 ± 16 | 111 ± 29 | 1.50 | 0.233 |
Root length (cm) | 22.3 ± 3.9 | 25.6 ± 1.7 | 23.2 ± 2.0 | 21.2 ± 3.8 | 24.3 ± 1.4 | 0.38 | 0.824 |
Root length/Container length (%) | 223 ± 40 c | 256 ± 17 c | 66 ± 6 ab | 106 ± 19 b | 54 ± 3 a | 16.06 | <0.05 |
Although there were no significant differences in root biomass and number of new roots, the distribution of these parameters varied with container depth (Fig. 2). Biomass and new roots were mainly concentrated at the location of the base of the first-stage root plug, except for T440-Short and T440-Long, in which the biomass and new roots were concentrated under the dotted line (Fig. 2). In Fig. 2, the point where the biomass curve intercepts the root number curve corresponds to 0.75 mg of biomass per root. When the root biomass curve is located to the left of the root number curve, the roots have a value less than 0.75 mg, which we define as “thin roots”. On the other hand, when the biomass curve is located to the right of the root number curve, the roots have a value greater than 0.75 mg, which we define as “thick roots”. Furthermore, it was observed that thick roots were concentrated under the dotted line and thin roots dominated above the dotted line (Fig. 2). In general, diverse criteria are used to define root size in plant studies. Generally, root diameter is the most common parameter to define size root classification. In some studies, thin or fine roots are defined as those less than 2 mm, while thick or coarse roots are defined as those greater than 2 mm ([16], [29]) or 4.5 mm ([32]) in diameter.
Fig. 2 - Distribution of new root biomass (white circles and lowercase letters) and new roots number (filled circles and capital letters) of Acacia caven seedlings for the root growth potential (RGP) test. Different letters indicate significant differences among treatments for each section of the container. The dotted line indicates the depth where the base of the first stage assay root plug was located.
Seedling roots of T440-C and T440-Short treatments colonized and were concentrated up to 30 cm in depth, while seedling roots of T880-Short and T880-Long treatments colonized up to 40 cm and 70 cm in depth, respectively. Seedling roots of T440-Long, which had half the volume of T880-Short and T880-Long, colonized up to 60 cm in depth (Fig. 2).
Discussion
The present study confirms that container characteristics have important implications on seedling morphology of deep-rooting species such as A. caven. Among the morphological variables influenced by type of container, a change in rooting volume distribution (short versus elongated container) was the most important, whereas container size (small versus large volume) had a minor influence on taproot length and RCD. Different plant responses to container type have been described by other authors for Mediterranean tree species ([8], [25]), and in many cases the differences depend on root growth strategies ([42]).
Field and experimental evidence has shown that direct sowing of tree species with a taproot system produces trees that can cope with water restrictions more effectively than those grown in nurseries and outplanted in field conditions, because of greater development of taproots ([50]). Therefore, elongated containers guarantee higher rates of seedling survival under semiarid conditions ([48]) as the root development mimics seedling growth under natural conditions. According to Peman et al. ([32]), water uptake efficiency could be seriously diminished by the confinement effect that plant roots suffer in short containers (20 cm in length). In addition, the same authors suggest that restrictions to vertical root growth of Quercus ilex result in total lower root lengths. In our study, we observed that elongated containers (35 to 45 cm in length), independent of their volume, promoted larger increases in taproot length than shorter containers. In fact, the largest container type (T880-Long) quintupled the length achieved in the smallest container (T440-Short). Similar results were found by Chirino et al. ([8]) using 30-cm-deep containers, where taproots reached 30 cm in length. In the same study, the 30-cm-deep containers were also associated with higher shoot and root biomass compared to the 18-cm container type.
In relation to the other plant quality attributes, the shoot height/RCD ratio values of all experimental treatments were greater than 100, indicating that the plants were very tall with regard to their RCD ([35]). Therefore, this type of plant may be considered too slender or insufficiently robust for semiarid environments ([28]). This response may be explained by the low level of photosynthetic active radiation (PAR) available in the plant growth room used for the present study, compared to the effects produced in plants cultivated under higher PAR found in Mediterranean areas ([43]). However, shorter containers promoted larger increases in RCD (increases of approximately 4 mm). Despite the short growing period of this study, the diameters obtained in our study meet the recommended value range for Mediterranean species seedlings ([28]).
Taproots were effectively pruned in all containers (T440-Short, T440-Long, T880-Short, and T880-Long) with basal pruning when they came into contact with air. Similar responses were reported by Mariotti et al. ([26]) using air-pots, where inhibited growth of Quercus ilex seedlings was obtained in deep pots. On the other hand, most A. caven seedlings grown in polyethylene bags (T440-C) showed spiral taproots at the base of the bag. When a plant develops a spiral root, it does not normally restart growth at depth after outplanting. Anchoring problems may occur ([12]), and seedlings may even die ([35]). It should also be noted that deformation of the root system can persist in the soil for years after outplanting ([23]).
In all treatments of the second stage of the experiment (RGP test), seedlings of A. caven were able to form new roots, indicating their potential capacity to develop roots out of the root plug once they are transplanted. Tsakaldimi et al. ([46]) observed for two Mediterranean evergreen oak species that seedlings restricting their roots to the root plug space do not survive once they are transplanted into soil. The RGP test showed that thinner roots developed on the sides of the root plug, which are potentially better surfaces for water absorption and nutrient uptake ([12]). Thicker root growth restarted at the base of the root plug, which improves anchorage and allows water absorption from deep soil layers. For example, in Q. suber plants. the largest concentration of biomass is located between 40 and 60 cm in depth ([8]), similar to the results of the RGP test with A. caven seedlings grown in elongated containers (T440-Long). The soil at 60 cm depth is significantly wetter than at 30 cm depth, which favours the survival of early growth stages under field conditions.
A minimum volume of 300 mL for propagation containers has been recommended for dry climates ([12], [34]). However, Del Campo et al. ([11]) found a negative effect on seedling quality in containers ranging from 250 to 300 mL, which resulted in an early halt to plant growth due to increased tissue lignification. On the other hand, larger containers (>300 mL) increase production costs as they require more substrate and occupy larger surfaces at the nursery ([25]). Larger containers also result in higher planting costs associated with increased soil movement ([34]), among others. Under these considerations and according to our findings, 440-mL containers are the most appropriate for the cultivation of A. caven seedlings. Specifically, the T440-Long container type has the greatest advantage as it has a smaller diameter and thus uses less space in the nursery.
Enhanced development of taproots without deformation at the container base is a key aspect before outplanting, as it assures resistance to severe and long drought periods in semiarid Mediterranean ecosystems. Therefore, our results suggest that adequate root development at early growth stages of seedlings would improve water-stress resistance under semiarid conditions, as also stated by Peman et al. ([32]). However, this needs to be evaluated under real field conditions. Some studies that evaluated post-transplant effects on seedlings grown in large-volume containers reported that those with greater availability of space for root growth resulted in larger height and diameter, better nutritional status, and higher survival rates ([12]). Therefore, the present study stresses the relevance of improving characteristics of propagation containers for xerophytic deep-rooting species destined for semiarid environments.
Conclusions
The rooting volume distribution of propagation containers positively affects taproot development of A. caven seedlings. Specifically, elongated containers (35 cm to 40 cm in length) with basal root self-pruning, such as T440-Long and T880-Long, produced seedlings with smaller shoot/root ratios, longer taproots, and greater ability to restart new root growth at deeper container layers. These results could be relevant for reconsidering container designs used at the nursery phase and thus expand alternatives for growers of xerophytic tree species with taproot systems.
Acknowledgements
The authors would like to thank the Chilean Research Center for Mining and Metallurgy (CIMM) and the CONICYT FB 0002 (2014) for funding the study; Professors Eduardo Olate and Paulina Fernández for their contribution to the present study; Elisabeth Trangolao, Elena Bustamante, Margaret Opazo and Marcela Jimenez for their help with the experiments; and Consuelo Gazitúa for helping with graphical presentations.
References
Gscholar
CrossRef | Gscholar
Gscholar
Gscholar
CrossRef | Gscholar
Gscholar
Gscholar
Gscholar
Gscholar
CrossRef | Gscholar
Authors’ Info
Authors’ Affiliation
Juan F Ovalle
Eduardo C Arellano
Rosanna Ginocchio
Pontificia Universidad Católica de Chile, Center of Applied Ecology & Sustainability (CAPES), PO Box 8331150, Santiago (Chile)
Rosanna Ginocchio
Pontificia Universidad Católica de Chile, Departamento de Ecosistemas y Medio Ambiente, Facultad de Agronomía e Ingeniería Forestal, PO Box 7820436, Santiago (Chile)
Corresponding author
Paper Info
Citation
De La Fuente LM, Ovalle JF, Arellano EC, Ginocchio R (2017). Use of alternative containers for promoting deep rooting of native forest species used for dryland restoration: the case of Acacia caven. iForest 10: 776-782. - doi: 10.3832/ifor2101-010
Academic Editor
Gianfranco Minotta
Paper history
Received: May 02, 2016
Accepted: Jun 15, 2017
First online: Sep 02, 2017
Publication Date: Oct 31, 2017
Publication Time: 2.63 months
Copyright Information
© SISEF - The Italian Society of Silviculture and Forest Ecology 2017
Open Access
This article is distributed under the terms of the Creative Commons Attribution-Non Commercial 4.0 International (https://creativecommons.org/licenses/by-nc/4.0/), which permits unrestricted use, distribution, and reproduction in any medium, provided you give appropriate credit to the original author(s) and the source, provide a link to the Creative Commons license, and indicate if changes were made.
Web Metrics
Breakdown by View Type
Article Usage
Total Article Views: 48360
(from publication date up to now)
Breakdown by View Type
HTML Page Views: 40472
Abstract Page Views: 3189
PDF Downloads: 3674
Citation/Reference Downloads: 17
XML Downloads: 1008
Web Metrics
Days since publication: 2873
Overall contacts: 48360
Avg. contacts per week: 117.83
Article Citations
Article citations are based on data periodically collected from the Clarivate Web of Science web site
(last update: Mar 2025)
Total number of cites (since 2017): 14
Average cites per year: 1.56
Publication Metrics
by Dimensions ©
Articles citing this article
List of the papers citing this article based on CrossRef Cited-by.
Related Contents
iForest Similar Articles
Research Articles
Conservation of Betula oycoviensis, an endangered rare taxon, using vegetative propagation methods
vol. 13, pp. 107-113 (online: 23 March 2020)
Technical Reports
De novo adventitious root formations in mini-cuttings of Azadirachta indica in response to different rooting media and auxin treatments
vol. 8, pp. 558-564 (online: 09 December 2014)
Research Articles
Controlled-release fertilizers combined with Pseudomonas fluorescens rhizobacteria inoculum improve growth in Pinus halepensis seedlings
vol. 8, pp. 12-18 (online: 12 May 2014)
Research Articles
Linking nursery nutritional status and water availability post-planting under intense summer drought: the case of a South American Mediterranean tree species
vol. 9, pp. 758-765 (online: 03 June 2016)
Short Communications
Effect of four levels of shade on survival, morphology and chlorophyll fluorescence of Nothofagus alessandrii container-grown seedlings
vol. 8, pp. 638-641 (online: 08 January 2015)
Technical Reports
Nursery practices increase seedling performance on nutrient-poor soils in Swietenia humilis
vol. 8, pp. 552-557 (online: 09 December 2014)
Research Articles
Forecasting the field performance of Austrian pine seedlings using morphological attributes
vol. 10, pp. 99-107 (online: 13 October 2016)
Research Articles
Seedling quality and short-term field performance of three Amazonian forest species as affected by site conditions
vol. 17, pp. 80-89 (online: 21 March 2024)
Research Articles
Fertilisation of Quercus seedlings inoculated with Tuber melanosporum: effects on growth and mycorrhization of two host species and two inoculation methods
vol. 10, pp. 267-272 (online: 13 December 2016)
Research Articles
The effect of calcium on the growth of native species in a tropical forest hotspot
vol. 11, pp. 221-226 (online: 01 March 2018)
iForest Database Search
Search By Author
Search By Keyword
Google Scholar Search
Citing Articles
Search By Author
Search By Keywords
PubMed Search
Search By Author
Search By Keyword