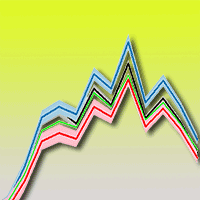
Prediction of ozone effects on net ecosystem production of Norway spruce forest
iForest - Biogeosciences and Forestry, Volume 11, Issue 6, Pages 743-750 (2018)
doi: https://doi.org/10.3832/ifor2805-011
Published: Nov 15, 2018 - Copyright © 2018 SISEF
Research Articles
Abstract
Future ground-level concentrations of phytotoxic ozone are projected to grow in the Northern Hemisphere, at a rate depending on emission scenarios. We explored the likely changes in net ecosystem production (NEP) due to the increasing concentration of tropospheric ozone by applying a Generalized Additive Mixed Model based on measurements of ozone concentration ([O3]) and stomatal ozone flux (FsO3), at a mountainous Norway spruce forest in the Czech Republic, Central Europe. A dataset covering the growing period (May-August 2009) was examined in this case study. A predictive model based on FsO3 was found to be marginally more accurate than a model using [O3] alone for prediction of the course of NEP when compared to NEP measured by the eddy covariance technique. Both higher [O3] and FsO3 were found to reduce NEP. NEP simulated at low, pre-industrial FsO3 (0.5 nmol m-2 s-1) was higher by 24.8% as compared to NEP assessed at current rates of FsO3 (8.32 nmol m-2 s-1). However, NEP simulated at high FsO3 (17 nmol m-2 s-1), likely in the future, was reduced by 14.1% as compared to NEP values at current FsO3. The interaction between environmental factors and stomatal conductance is discussed in this paper.
Keywords
Introduction
Tropospheric ozone (O3), a common phytotoxic secondary air pollutant, reduces growth and carbon sequestration potential of terrestrial vegetation ([34]). Despite the limited nature of observations in the 19th century, ozone concentrations are reported to have undergone an increase from the base level of 10-15 ppbv in the pre-industrial era ([28]) to current concentrations of 35-45 ppbv in large parts of the industrialised world, with an average rate of increase of about 5 ppbv per decade in the Northern Hemisphere ([7]). It is estimated that the long-term increase in tropospheric O3 concentration ([O3]) has led to a substantial reduction in carbon uptake, as compared to the pre-industrial era ([7]). While daily maxima of [O3] may now be decreasing, annual mean [O3] has been increasing over the last decade ([23]).
Recent studies at different hierarchical levels have shown that O3 reduces photosynthetic carbon assimilation and changes carbon allocation in trees and forest ecosystems ([41], [30]). It has been demonstrated that O3 alters the source-sink balance in plants, resulting initially in carbon retention in shoots and decreased carbon allocation in below ground biomass (reviewed by [2]). Rising [O3] thus has a significant potential to affect terrestrial carbon sinks and regional hydrology ([41], [35]). Most of the studies were, however, conducted on seedlings or juvenile trees and the assessment of the O3 impact on mature trees is still at an early stage ([47], [48], [9]). Accordingly, there is an increasing demand for long-term studies conducted under natural, ecologically relevant conditions ([10]).
To quantify the detrimental dose of O3 on vegetation, several indices have been established: AOT40 (accumulated exposure over a threshold of 40 ppbv) and PODy (accumulated ozone flux above a given flux threshold y [nmol m-2 s-1]). Critical levels are assessed as an estimation above which detrimental effects may occur. The magnitude of both indices depends on species, environmental conditions and the capacity of plant defence mechanisms ([22]). Verryckt et al. ([38]) reported no impact of ambient [O3] on carbon uptake in sub-urban mature pine forest in the vicinity of Antwerp city, Belgium, although the critical levels for both AOT40 and POD1 indices were exceeded. Similarly, an ambient [O3] of 50 ppbv (mean value) had no effect on leaf injury in black cherry and red maple seedlings during the period of April-August. While visible symptoms of foliar injury were observed under doubled [O3] ([29]), no visible damages of leaves nor reduction in CO2 uptake were observed in poplar plantation at a cumulative stomatal O3 uptake of 25-27 mmol m-2 ([49]).
Contrary to these findings, growth decreased by 6.6% in Swiss forests during the period 1991-2011, particularly in European beech and Norway spruce ([5]), and this decline was ascribed to rising [O3]. Similarly, Gross Primary Production (GPP) was reduced by 4-8% on average in eastern US vegetation peaking up to 11-17% during an occurrence of high O3 episodes ([45]). Only a limited effect of O3 on photosynthesis was observed in the broad-leaved Harvard forest ([44]). Norway spruce (Picea abies Karst.), a wide-spread tree species of the temperate zone and especially Central Europe, has previously been described as an ozone sensitive tree species ([47]). Subramanian et al. ([34]), among others, reported a decrease in photosynthetic carbon assimilation of 4.3-15.5% due to an exposure to high [O3]. As most studies were conducted on seedlings under controlled conditions ([32]), estimation and quantification of O3 effects at the ecosystem scale under field conditions received only limited attention.
In the present study, we attempted to quantify the effect of O3 on Net Ecosystem Production (NEP), defined as the difference between ecosystem-level photosynthetic uptake of CO2 and ecosystem respiratory loss of CO2. Our attention was focussed on a mountainous spruce forest in the Czech Republic. NEP values measured by eddy covariance were compared to those estimated by the Generalized Additive Mixed Model (GAMM). We tested the efficacy of [O3]- and stomatal ozone flux (FsO3)-based GAMM models to predict diurnal and seasonal changes in NEP. To determine O3 effects, NEP was calculated in a simulation model at low, pre-industrial [O3] (12 ppbv) and FsO3 (0.5 nmol m-2 s-1) as well as at high [O3] (80 ppbv) and FsO3 (17 nmol m-2 s-1) expected at the end of the century ([21], [7]). We hypothesized that tropospheric ozone reduces NEP in Norway spruce forest. To test this hypothesis, we investigated effects of [O3] and FsO3 on NEP during four representative days of the main growing season differing in degree of cloudiness and thus in daily maxima of solar radiation intensity, air temperature, and vapour pressure deficit. These representative days also covered seasonal course in NEP and enabled thus to model effects of [O3] and FsO3 on NEP throughout the growing season (May-August).
Material and methods
Site description
The forest stand is located at the Bílý Kríž experimental research site within the Beskydy Mountains in the north-east of the Czech Republic (49° 30′ N, 18° 32′ E; 875-908 m a.s.l.). The experimental research site forms part of several international research networks and infrastructures: CzeCOS (Czech Carbon Observation System), ICOS (Integrated Carbon Observation System), and AnaEE (Analysis and Experimentation on Ecosystems). The forest stand (99% Picea abies and 1% Abies alba) had been established in 1981 by row planting of 4-year-old Norway spruce seedlings. Mean stand slope is 13° and exposure is to the south. In 2009, the year of investigation, the average stand height was 13 m, tree density was 1420 trees ha-1, average stem diameter at breast height was 15.9 cm, and leaf area index based on hemispherical photographs was 9.6 m2 m-2.
The area has a moderately cool (mean air temperature 6.8 °C) and humid (mean relative air humidity 84%) climate with high annual precipitation (mean annual precipitation 1318 mm; years 1998-2009). Due to an even distribution of precipitation over the year, values of soil volumetric water content remain high during the growing season ranging between 20 and 30% irrespective of sky conditions. The region is characterized by low concentrations of nitrogen oxides (below 10 ppbv) and high [O3], exceeding 80 ppbv during summer months ([47]). Diurnal courses of selected environmental variables, including diurnal changes in [O3], for representative days are shown at Fig. 1.
Fig. 1 - Diurnal courses of global radiation (A), air temperature (B), vapour pressure deficit (VPD, C), and ozone concentration (D) during the representative days.
Measurement of environmental variables
The measurements were conducted from May 28 to September 30, 2009, during the daytime (06:00-18:00 GMT+1) to cover the periods characterized by well-developed turbulent mixing. A meteorological mast (36 m tall) situated within the studied stand was equipped with a set of meteorological sensors and an eddy covariance system. Air temperature (Tair) and relative air humidity (RH) were measured by an EMS33 Rotro® sensor (EMS, Brno, Czech Republic). These variables were used to calculate the values of vapour pressure deficit (VPD) according to the formula (eqn. 1):
The barometers PTB110® (Vaisala, Vantaa, Finland) and SPA 511 B5UB® (CRESSTO, Roznov pod Radhostem, Czech Republic) were used to measure air pressure. Global radiation (GR) was measured by a pyranometer CM6B® (Kipp & Zonen, Delft, Netherlands). The 2D ultrasonic anemometer 50.5 (Met One Instruments, Grants Pass, OR, USA) was used for the measurement of horizontal wind speed. All standard meteorological measurements were made on a vertical profile at heights of 2, 7, 10, 11.5, 12.25, 13, 13.5, 14, 15, 17, 21, and 28 m above the soil surface. The signals from all sensors were recorded every 30 s and stored as half-hourly averages using a data logger (Delta-T®, Burwell, Cambridgeshire, UK). In addition, precipitation was recorded by a precipitation gauge 386C (Met One Instruments, Grants Pass, OR, USA).
Estimation of CO2 and O3 fluxes
An eddy covariance system was used to measure the CO2 and water vapour fluxes between the forest stand and the atmosphere. The system consisted of a Gill R3® ultrasonic anemometer (Gill Instruments, Hampshire, UK) and an enclosed-path infrared gas analyser LI-7200® (LI-COR Biosciences, Lincoln, NE, USA) placed 18 m above the soil surface. The post-processing of high frequency data (20 Hz) was performed by EddyPro® software (LI-COR Biosciences, USA) according to recent recommendations ([3]) and expressed as half-hourly estimates. This procedure included spike removal and quality check of the raw signals, rotation of wind velocity components into the planar fit coordinate system, and spectral corrections of computed fluxes. The missing and excluded data, based on the quality checking scheme, were gap-filled by the marginal distribution sampling method according to Reichstein et al. ([25]). Sign convention was adopted as follows: positive values represent CO2 uptake, thus enabling convenient modelling of NEP as a general nonrectangular hyperbolic function of incident GR ([37]).
[O3] was measured at 5, 15, and 25 m above the soil surface using slow-response O3 analysers O341M® (Environment S.A., Poissy, France). The signals from all O3 analysers were recorded as half-hourly averages.
Stomatal ozone flux (FsO3) to the Norway spruce forest was calculated according to Cieslik ([6] - eqn. 2)
where c(z) is O3 concentration at a height z = 2 m above the canopy, Ra is the aerodynamic resistance for the turbulent layer, Rb is the laminar layer resistance for the quasi-laminar layer, and Rc is the surface or canopy resistance of the receptor. Rc was calculated as (eqn. 3):
where Rsto is the land-cover specific needle stomatal resistance to O3 uptake, Rext is the resistance of the external plant parts to uptake or destruction of O3, Rinc is the land-cover specific in-canopy aerodynamic resistance to transport of O3 towards the soil and lower parts of the canopy, Rsoil is the soil resistance to destruction or absorption of O3 at the ground surface, LAI is leaf area index, and SAI is a surface area index set equal to LAI in the growing season.
Stomatal resistance component Rsto was calculated as described in Emberson et al. ([8] - eqn. 4):
where gmax is the average maximum stomatal conductance of Picea abies to ozone (nmol O3 m-2 s-1) expressed on total needle surface area. The parameters gphen, glight, gtemp, gVPD, and gSWP are expressed in relative terms between 0 and 1, and represent the modification of gmax due to phenological changes, light intensity, air temperature, vapor pressure deficit, and soil water potential, respectively. gmin is the minimum FsO3 that occurs during daylight hours.
The model was applied using environmental variables measured at the experimental station, although soil moisture deficit was estimated as a function of precipitation and daily mean surface temperature according to the principles of water budget. For more details and the site-specific model parameterisation see Zapletal et al. ([47]).
Modelling and statistical analyses
The Generalized Additive Mixed Model (GAMM) was implemented in the “mgcv” package of the R ver. 3.4.0 program ([42]) to assess the dependencies between NEP and its predictors GR, RH, Tair, VPD, and transpiration rate along with [O3] and FsO3 (Tab. 1). One-hour averages of all input data were used in GAMM. Only the data of the daytime period (06:00-18:00) when GR exceeded 10 W m-2 were used, so as to exclude periods where turbulence was not well-developed.
Tab. 1 - Meteorological variables of the period May 28-September 30, 2009. [O3]: ozone concentration; (FsO3): stomatal ozone flux; (VPD): vapour pressure deficit; (GR): global radiation; (RH): relative air humidity; (Tair): air temperature; (Tr): transpiration; (NEP): net ecosystem production: (SD): standard deviation.
Variable | Unit | Mean | SD | Range |
---|---|---|---|---|
NEP | µmol m-2 s-1 | 12.1 | 9.2 | -7.9 - 31.7 |
[O3] | ppbv | 42.9 | 11.2 | 12.0 - 79.5 |
FsO3 | nmol m-2 s-1 | 8.3 | 3.6 | 0.072 - 17.6 |
GR | W m-2 | 342.3 | 247.4 | 0.86 - 974 |
Tair | °C | 17.7 | 6.1 | 1.3 - 33.8 |
RH | % | 75.2 | 17.3 | 37.6 - 99.9 |
Tr | mm h-1 | 0.0878 | 0.0754 | 0 - 0.37 |
VPD | kPa | 0.624 | 0.585 | 0.0006 - 2.89 |
All predictors were tested and fitted by a linear model, in the form of splines as smooth functions and as 1st degree linear interactions. Predictors were centred and, in the case of GR and RH, a square root transformation was used. Day of observation and autocorrelation pattern were tested as random factors. The best model was selected based on the AIC criteria and p-values. The model was started as one component containing all explanatory variables together with their interactions. By use of REML (Restricted Maximum Likelihood Estimation) the optimal structure of the random component was found. Then, optimal fixed structure using ML (Maximum Likehood) estimation was determined. Within the fixed structure, REML estimation was applied again.
Results
Microclimate conditions, [O3] and FsO3
The mean incoming daily sum of GR was 15.8 MJ m-2, mean Tair was 15.03 °C, RH was 81.5%, and precipitation sum was 388 mm during the investigated period from May 28 to September 30. Soil volumetric water content remained high during the whole growing season ranging between 20 and 30% irrespective of actual sky conditions. Four model days, selected on the base of cloudiness degree (Fig. 1), represent (i) a sunny warm day with high Tair and VPD values (July 22) and (ii) partly-cloudy sky conditions with mild Tair and VPD (June 17 and August 16). (iii) The onset of the growing season (May 30) was characterized by the minimum values of GR, Tair and VPD. These days were also characterized by distinct [O3] with daily maxima ranging between 38 and 64 ppbv (Fig. 1D) as well as diurnal courses of FsO3 (Fig. S1 in Supplementary material). The most frequent FsO3 values ranged from 8-12 nmol m-2 s-1 during sunlight hours when the whole dataset is considered (Fig. 2).
Fig. 2 - Relative occurrence of stomatal ozone flux (FsO3) during the sunlight hours modelled for the period May 28-September 30, 2009.
GAMM outputs
The results of GAMM model for NEP prediction including [O3] and FsO3 are shown in Tab. 2 and Tab. 3, respectively. Both models include a linear and non-linear part, as illustrated by the Edf parameter, which is presented only in the non-linear part. Only statistically significant predictors are shown. [O3] and VPD variables are shown only in Tab. 2, as their effect on NEP was only linear. Based on the Edf parameter, it was shown that FsO3 had a non-linear effect on NEP (Tab. 3).
Tab. 2 - Outputs of Generalized Additive Mixed Model (GAMM) predicting mixed effects of [O3] and other selected micrometeorological variables (predictors) on net ecosystem production (NEP). Linear predictors and linear interaction statistics are shown as t-values and p-values, whereas statistics of predictors used as smooth terms are shown as F-statistics, p-values and Edf. × indicate interaction. (Edf): estimated degree of freedom, Edf >1 indicate nonlinear relationships. R2 (adjusted) = 0.948, n = 1440. [O3]: ozone concentration; (VPD): vapour pressure deficit; (GR): global radiation; (RH): relative air humidity; (Tair): air temperature; (Tr): transpiration (***): p<0.001; (**): p<0.01; (*): p<0.05.
Predictor/ Interaction |
Goodness of fit | Statistics | |||
---|---|---|---|---|---|
Parametric estimate |
Edf | t-value | F | p-value | |
[O3] | -0.042609 | - | 4.655 | - | 3.55e-06*** |
VPD | 1.686854 | - | 2.226 | - | 0.026154* |
[O3] × GR | -0.002573 | - | -3.390 | - | 0.000717*** |
GR × RH | -0.100322 | - | -6.310 | - | 3.73e-10*** |
GR × Tair | 0.013316 | - | 6.919 | - | 6.84e-12*** |
GR × Tr | -0.476992 | - | -3.499 | - | 0.000481*** |
VPD × GR | -0.280066 | - | -8.314 | - | <2e-16*** |
s(GR) | - | 2.987 | - | 6835.670 | <2e-16*** |
s(RH) | - | 2.878 | - | 22.424 | 6.5e-13*** |
s(Tair) | - | 2.4577 | - | 6.775 | 0.000807*** |
s(Tr) | - | 2.384 | - | 5.168 | 0.003775** |
Tab. 3 - Outputs of Generalized Additive Mixed Model (GAMM) predicting mixed effects of stomatal ozone flux (FsO3) and other selected micrometeorological variables (predictors) on net ecosystem production (NEP). Linear predictors and linear interaction statistics are shown as t-values and p-values, whereas statistics of predictors used as smooth terms are shown as F-statistics, p-values and Edf. × indicate interaction. (Edf): estimated degree of freedom; Edf values >1 indicate nonlinear relationships. R2 (adjusted) = 0.95, n = 1440. (FsO3): stomatal ozone flux; (VPD): vapour pressure deficit; (GR): global radiation; (RH): relative air humidity; (Tair): air temperature; (Tr): transpiration; (***): p<0.001; (**): p<0.01; (*): p<0.05.
Predictor/ Interaction |
Goodness of fit | Statistics | |||
---|---|---|---|---|---|
Parametric estimate |
Edf | t-value | F | p-value | |
VPD | 2.301725 | - | 2.850 | - | 0.00443** |
GR × FsO3 | -0.016042 | - | -4.577 | - | 5.14e-06*** |
GR × RH | -0.142519 | - | -7.082 | - | 2.23e-12*** |
GR × Tair | 0.014084 | - | 7.378 | - | 2.73e-13*** |
VPD × GR | -0.388303 | - | -9.721 | - | <2e-16*** |
s(GR) | - | 2.973 | - | 6314.858 | <2e-16*** |
s(RH) | - | 2.865 | - | 18.079 | 5.99e-08*** |
s(Tair) | - | 2.824 | - | 11.175 | 8.78e-07*** |
s(FsO3) | - | 2.933 | - | 22.547 | 7.77e-13*** |
s(Tr) | - | 2.701 | - | 7.112 | 0.000131*** |
Statistically significant (p < 0.01) interactions were found between [O3] and GR and between FsO3 and GR (Tab. 2, Tab. 3). Both interactions had negative values of parametric estimate (Tab. 2, Tab. 3) indicating that [O3] and FsO3 led to a larger reduction of NEP with increasing GR. For example, natural FsO3 led to a decrease in NEP by 1.07 µmol m-2 s-1, comparing to pre-industrial era, at GR <300 W m-2, whereas this reduction amounted up to 1.59 µmol m-2 s-1 (23.6%) at GR >500 W m-2. The FsO3 seems to be more tightly linked to ozone-induced decline of NEP than [O3] itself, based on explained variance by the models (R2) and more significant p-value for FsO3 (p = 7.77e-13) that [O3] (p = 3.55e-06). GR was identified as the most important driver of NEP, having the most significant p values (Tab. 2, Tab. 3).
NEP modelling
NEP was predicted using [O3] (Fig. 3A) and FsO3 (Fig. 3B) values in the range from zero to maximal values determined during the investigated growing season (May-September). Values of other predictors were fixed to values measured at 11:00 of the representative days. Both models predict a decrease in NEP with increasing [O3] or increasing FsO3. Among the representative days, the highest decrease of NEP was found on May 30th, amounting to 21.6% (model run with [O3]) and 30.4% (model run with FsO3) when compared NEP at minimum (affected by 12 ppbv and 0.5 nmol m-2 s-1) and maximum (affected by 80 ppbv and 17 nmol m-2 s-1) values of [O3] and FsO3, respectively. In July 22, the warmest month, the corresponding decreases were 24.9% and 28.9%. Diurnal courses of measured and modelled NEP during four representative days of the vegetation season are shown in Fig. 4.
Fig. 3 - Modelled relationships between net ecosystem production (NEP) and ozone concentration (A) and between NEP and stomatal ozone flux (B) for four representative days (full line). Models were applied with fixed predictors (see Tab. 1) measured at 11:00 of these representative days. Dashed lines represent the 95% confidence interval.
Fig. 4 - Predicted diurnal courses of net ecosystem production (NEP) using Generalized Additive Mixed Model with ozone concentration (O3, A,B,C,D) and stomatal ozone flux (FsO3, E,F,G,H). Three levels of O3 and FsO3 were used to predict NEP: Blue line - low O3 (10 ppbv) and FsO3 (0.5 nmol m-2 s-1), red line - high O3 (80 ppbv) and FsO3 (17 nmol m-2 s-1), and black line - actual, measured O3 and FsO3. Green line represents NEP measured by an eddy covariance system. Shaded area represents prediction bounds at 95% confidence level.
Multi-factorial analysis (Fig. S2 in Supplementary material) revealed that high FsO3 (17 nmol m-2 s-1) has the most significant effect on NEP reduction in summer, while NEP decrease is driven more by GR than FsO3 in spring and autumn. Moreover, VPD-induced stomatal closure was a main factor limiting NEP and stomatal O3 flux in Norway spruce trees throughout the whole growing season. Therefore, a strong relationship between NEP reduction and stomatal conductance, not only the simple [O3] outside the leaves, can be suggested.
Taking to the account the whole dataset, the GAMM based calculated NEP was higher by up to 12.4% at low, preindustrial ozone concentration (12 ppbv) as compared to the measured NEP at the present concentration of 42.9 ± 11.2 ppbv (mean ± SD - Tab. 1). Similarly, the GAMM based on FsO3 revealed NEP to be elevated by up to 24.8% under the condition of low FsO3 (0.5 nmol m-2 s-1) as compared to actual FsO3 of 8.3 ± 3.7 nmol m-2 s-1 (mean ± SD). In contrast, high [O3] (80 ppbv) and high FsO3 (17 nmol m-2 s-1) were found to lead to a decrease in NEP by 24% and 38.9%, respectively as compared to preindustrial NEP values which were unaffected by high [O3] and/or FsO3 (Tab. 4).
Tab. 4 - Mean values of ambient (Amb) ozone concentration ([O3]; ppbv) and stomatal ozone flux (FsO3; nmol m-2 s-1), and Net ecosystem production (NEP; µmol m-2 s-1) predicted by a Generalized Additive Mixed Model for the conditions of actual (measured), high, and low [O3] and FsO3 during the individual months of the growing season and the whole dataset covering May 28-September 30, 2009. Δ represents percentage difference of predicted NEP to mean NEP measured by an eddy covariance system. (a) values of Δ represent percentage decrease in NEP induced by high O3 (80 ppbv) and/or FsO3 (17 nmol m-2 s-1); (b): values of Δ represent percentage increase in NEP at low O3 (12 ppbv) and/or FsO3 (0.5 nmol m-2 s-1).
Months | Conditions | Amb [O3] | Low [O3] | High [O3] | Amb FsO3 | Low FsO3 | High FsO3 |
---|---|---|---|---|---|---|---|
May | [O3]/FsO3 | 36.5 | 12 | 80 | 5.5 | 0.5 | 17 |
NEP | 7.9 | 9.6 | 7.2 | 7.9 | 10.8 | 7.2 | |
Δ | - | 21.5 b | 8.9 a | - | 36.7 b | 8.9 a | |
June | [O3]/FsO3 | 42 | 12 | 80 | 9.1 | 0.5 | 17 |
NEP | 10.2 | 12.5 | 9.7 | 10.2 | 14.1 | 7.7 | |
Δ | - | 22.5 b | 4.9 a | - | 38.2 b | 24.5 a | |
July | [O3]/FsO3 | 46.2 | 12 | 80 | 8.98 | 0.5 | 17 |
NEP | 15.3 | 17.2 | 13.8 | 15.3 | 18.7 | 13.5 | |
Δ | - | 12.4 b | 9.8 a | - | 22.2 b | 11.8 a | |
Aug | [O3]/FsO3 | 45 | 12 | 80 | 8.5 | 0.5 | 17 |
NEP | 12.6 | 13.9 | 11 | 12.6 | 15.4 | 10.9 | |
Δ | - | 10.3 b | 12.7 a | - | 22.2 b | 13.5 a | |
Sept | [O3]/FsO3 | 39.1 | 12 | 80 | 7.2 | 0.5 | 17 |
NEP | 10.4 | 10.8 | 8.3 | 10.4 | 12.3 | 8.5 | |
Δ | - | 3.8 | 20.2 | - | 18.3 | 18.3 | |
Whole dataset | [O3]/FsO3 | 42.9 | 12 | 80 | 8.3 | 0.5 | 17 |
NEP | 12.1 | 13.6 | 10.7 | 12.1 | 15.1 | 10.4 | |
Δ | - | 12.4 | 11.6 | - | 24.8 | 14.1 |
In addition, we present estimated changes in NEP under the conditions of low/high [O3] and FsO3 during individual months of the growing season (May-September - Tab. 4). Both models confirmed a high percentage reduction of NEP occurring in spring (May-June), comparing values of current NEP to preindustrial ones, while the O3-induced NEP reduction in September was relatively small. The largest absolute reduction occurred, however, in June and July when NEP reaches a seasonal maximum. Contrary to those findings, the largest percentage and absolute NEP reductions predicted at high [O3] and FsO3 were found in September whereas the lowest ones in June.
Discussion
Projections of O3-induced damage are uncertain due to numerous scenarios dealing with different future [O3] or FsO3. Ozone in the Northern Hemisphere is projected to increase further by 20-25% between 2015 and 2050, and by 40-60% by 2100, if current ozone-precursors (CO2, volatile organic compounds, NOx) emission trends continue ([21]). Sitch et al. ([31]) projected a decrease in NEP by 14-23% over the period 1901-2100 owing to plant damage caused by [O3] with regional reduction peeking up to 30%. However, recent studies suggest [O3] decreases on average by 0-2 ppbv between 2000-2030 years, rising to 10 ppbv in 2100 in north latitudes ([43]). Those findings are in accordance with Klingberg et al. ([18]), who expected a decrease of O3 precursors, particularly biogenic volatile organic compounds (BVOCs) and nitrogen oxides (NOX), according to RCP4.5 scenario, which should consequently lead to decrease of European vegetation damage. However, extreme periods expected under future climate conditions may lead to episodes with high [O3]. For example, severe drought conditions in 2003 were associated with high [O3] in the Central Europe ([33]). In relation to regional climate, Beniston ([4]) assumed that the year 2003 could be used as an analogy of future dry summer seasons resulting in high emissions of BVOCs and NOX ([43]), which showed to depend highly on season also at Bílý Kríž ([15]). Future impacts on ozone concentration across Europe show contradicting results: under RCP8.5 scenario, [O3] of temperate coniferous forest could increase by 10.2 ppbv as modelled for coniferous forests at Hengduan Shan, China forests ([12]). On the other hand, a modelling study for the temperate coniferous forest of the Sierra Nevada suggests a decrease in [O3] by 6 ppbv from 2000 to 2050 ([12]) in accordance with the [O3] decrease by 5 ppbv per decade ([7]). In our study we have contrasted the O3 effects on NEP at low, pre-industrial [O3] of 12 ppbv and high [O3] of 80 ppbv.
Numerous studies have shown that elevated [O3] generally results in reduced photosynthesis, chlorophyll content and whole-plant growth, decreased stomatal conductance, an altered antioxidant system, accelerated senescence and changes the plant metabolism, although the extent of the effects varies by species, length of exposure, [O3] and/or co-occurrence of other stress factors (reviewed in [39], [19], [14]). In accordance with these studies we have found here that both increasing [O3] and FsO3 significantly reduced NEP of mature Norway spruce forest grown under natural mountain conditions (Fig. 3). Moreover, our analyses show that the use of FsO3 provides an improved fit to NEP experimental data as compared to the predictions of a merely [O3]-based GAMM model, particularly under hot summer days (Tab. 4). Differences between the measured and predicted NEP by the model with [O3] and FsO3 expressed as a model error amounted to 0.41% and 0.17%, respectively. The theory behind this is that stomata substantially regulate both CO2 and O3 diffusion into the leaf interior leading thus to a modulation of photosynthetic activity and the magnitude of O3-induced injuries, respectively. We assume that stomata remain open under the conditions of low VPD and sufficient light intensity ([37]). Accordingly, high FsO3 can be expected though ambient [O3] is relatively low and can lead to a substantial NEP reduction. On the contrary, conditions of high VPD and/or high temperature may result in a marked closure of stomata. While an ambient [O3] is usually high under such conditions, O3-induced reduction of NEP may be depressed due to a low FsO3 into a leaf interior. Therefore, models with high FsO3 may predict higher reduction in NEP than models with high [O3] (Fig. 3). Such results imply that not only high [O3], but particularly high FsO3 induces injuries on vegetation and reduces carbon assimilation ([20], [9]).
We predicted higher NEP (by 24.6% on average) at low FsO3 of 0.5 nmol m-2 s-1 as compared to NEP at current FsO3 of 8.32 ± 3.66 nmol m-2 s-1 with the highest absolute reduction occurring in June and July (Tab. 4). Karlsson ([16]) stated that reduction of the living biomass carbon stock caused by the ozone problem among the Central and Northern European countries ranges from 2% (Norway, Finland) to 32% in the Czech Republic, when compared to the pre-industrial age. Satellite data over the European continent suggest a decline of photosynthetic carbon uptake ranging between 0.4-30% due to O3 damage depending on the forest type and location. Highest negative effects of O3 were found in coniferous forests ([24]). Moreover, these authors identified air temperature, soil water content, and relative air humidity as key predictors of NEP describing more than 81% of NEP variability. Soil water content was, however, omitted into our model, since soil water content was not a limiting factor in our case study under the wet mountainous conditions. However, air temperature and relative air humidity (ultimately VPD), play significant roles in our model together with GR. Similarly to our findings, Anav et al. ([1]) reported a reduction of photosynthetic carbon uptake per day caused by [O3] of about 22% in boreal and temperate forests. FACE fumigation experiment of enhanced CO2 and [O3] on temperate forest dominated by Betula papyrifera Marsh. and Acer saccharum Marsh. in USA revealed reduction of cumulative net primary productivity of 9% ([36]). However, Finco et al. ([11]) estimated relatively low decreases in biomass production by 4-5 % in Alpine larch forest caused by current [O3] when compared to the pre-industrial era, even if the total ozone fluxes were generally high, up to 30-40 nmol m-2 s-1. This was due to relatively low portion of stomatal uptake by the larch forest representing only 15-16% of total O3 deposition flux into the forest. Similar growth reductions in stem increment of 6.6% and photosynthetic carbon uptake of 4-8% were observed in Switzerland ([5]) and eastern parts of USA ([45]), respectively, due to episodes of high tropospheric [O3].
Norway spruce, one of the most common and important timber trees in Europe, was found to be an O3 sensitive tree species, showing substantial reductions in photosynthetic carbon uptake under elevated [O3] ([39], [47]). For example, Subramanian et al. ([34]) reported a decrease in carbon sequestration of spruce forest in Sweden by 4.3-15.5% under ambient ozone conditions as compared to the preindustrial [O3]. This is consistent with our estimate of NEP reduction in mountain spruce forest of the temperate zone.
It should be noted, however, that there is a contrasting evidence of long-term O3 effects detected after several growing seasons. Since several research groups reported no negative effect of elevated [O3] on carbon assimilation in forest ecosystems ([46], [40]), we can hypothesize large acclimation capacity of tree species, including morphological ([26], [27]) as well as biochemical adjustments (reviewed by [13]). Recent studies have, however, shown that long-term exposure to elevated [O3] may substantially modulate an acclimation of trees to other environmental factors like elevated temperature ([17]) and/or elevated atmospheric CO2 concentration ([27], [46]). The carbon sink strength in old forests could be thus reduced substantially or even disappear under the conditions of high [O3], accelerating the impact of atmospheric CO2 on climate change. Precise estimates of FsO3 are thus needed for future predictions of ozone impacts on NEP of terrestrial ecosystems.
Conclusions
We have found that stomatal ozone flux model tracks diurnal changes in NEP more precisely that ozone concentration model does. NEP simulated at low, pre-industrial FsO3 (0.5 nmol m-2 s-1) was higher by 24.8% as compared to NEP assessed at current FsO3 (8.32 nmol m-2 s-1). Further increase in FsO3 (up to 17 nmol m-2 s-1) may lead to subsequent reduction in NEP by 14.1% in average as compared to current NEP values, but this reduction may change during the growing season. These results are in agreements with observations in other European coniferous forests. Relatively high site-specific variability in [O3] effect of photosynthetic carbon uptake is likely caused by species-specific sensitivity of stomata to environmental drivers. Also a co-occurrence of other stress factors, particularly extreme temperatures and drought, leading to the changes in stomatal conductance and stomatal ozone flux, may influence a final response of trees to [O3].
Acknowledgement
This work was supported by the Ministry of Education, Youth and Sports of the Czech Republic within the project “SustES - Adaptation strategies for sustainable ecosystem services and food security under adverse environmental conditions” (CZ.02. 1.01/0.0/0.0/16_019/0000797).
References
CrossRef | Gscholar
CrossRef | Gscholar
CrossRef | Gscholar
CrossRef | Gscholar
Gscholar
CrossRef | Gscholar
CrossRef | Gscholar
CrossRef | Gscholar
CrossRef | Gscholar
CrossRef | Gscholar
Authors’ Info
Authors’ Affiliation
Magda Edwards-Jonášová
Pavel Cudlín
Miloš Zapletal
Ladislav Šigut
John Grace
Otmar Urban
Global Change Research Institute CAS, Belidla 986/4a, 603 00 Brno (Czech Republic)
Silesian University in Opava, Faculty of Philosophy and Science, Masarykova 37, 746 01 Opava (Czech Republic)
University of Edinburgh, School of GeoSciences, Crew Bldg, Kings Bldgs, Alexander Crum Brown Rd, Edinburgh EH9 3FF, Midlothian (United Kingdom)
Corresponding author
Paper Info
Citation
Jurán S, Edwards-Jonášová M, Cudlín P, Zapletal M, Šigut L, Grace J, Urban O (2018). Prediction of ozone effects on net ecosystem production of Norway spruce forest. iForest 11: 743-750. - doi: 10.3832/ifor2805-011
Academic Editor
Silvano Fares
Paper history
Received: Apr 04, 2018
Accepted: Aug 27, 2018
First online: Nov 15, 2018
Publication Date: Dec 31, 2018
Publication Time: 2.67 months
Copyright Information
© SISEF - The Italian Society of Silviculture and Forest Ecology 2018
Open Access
This article is distributed under the terms of the Creative Commons Attribution-Non Commercial 4.0 International (https://creativecommons.org/licenses/by-nc/4.0/), which permits unrestricted use, distribution, and reproduction in any medium, provided you give appropriate credit to the original author(s) and the source, provide a link to the Creative Commons license, and indicate if changes were made.
Web Metrics
Breakdown by View Type
Article Usage
Total Article Views: 44980
(from publication date up to now)
Breakdown by View Type
HTML Page Views: 37744
Abstract Page Views: 3372
PDF Downloads: 3007
Citation/Reference Downloads: 8
XML Downloads: 849
Web Metrics
Days since publication: 2421
Overall contacts: 44980
Avg. contacts per week: 130.05
Article Citations
Article citations are based on data periodically collected from the Clarivate Web of Science web site
(last update: Mar 2025)
Total number of cites (since 2018): 19
Average cites per year: 2.38
Publication Metrics
by Dimensions ©
Articles citing this article
List of the papers citing this article based on CrossRef Cited-by.
Related Contents
iForest Similar Articles
Research Articles
A comparison between stomatal ozone uptake and AOT40 of deciduous trees in Japan
vol. 4, pp. 128-135 (online: 01 June 2011)
Short Communications
Ozone flux modelling for risk assessment: status and research needs
vol. 2, pp. 34-37 (online: 21 January 2009)
Research Articles
Effects of abiotic stress on gene transcription in European beech: ozone affects ethylene biosynthesis in saplings of Fagus sylvatica L.
vol. 2, pp. 114-118 (online: 10 June 2009)
Research Articles
Changes in the proteome of juvenile European beech following three years exposure to free-air elevated ozone
vol. 4, pp. 69-76 (online: 05 April 2011)
Research Articles
Soil drench of ethylenediurea (EDU) protects sensitive trees from ozone injury
vol. 4, pp. 66-68 (online: 05 April 2011)
Research Articles
Ozone fumigation effects on the morphology and biomass of Norway spruce (Picea abies L.) saplings
vol. 2, pp. 15-18 (online: 21 January 2009)
Research Articles
Testing a dual isotope model to track carbon and water gas exchanges in a Mediterranean forest
vol. 2, pp. 59-66 (online: 18 March 2009)
Research Articles
Ambient ozone phytotoxic potential over the Czech forests as assessed by AOT40
vol. 5, pp. 153-162 (online: 25 June 2012)
Research Articles
A new approach to ozone plant fumigation: The Web-O3-Fumigation. Isoprene response to a gradient of ozone stress in leaves of Quercus pubescens
vol. 1, pp. 22-26 (online: 28 February 2008)
Review Papers
Monitoring the effects of air pollution on forest condition in Europe: is crown defoliation an adequate indicator?
vol. 3, pp. 86-88 (online: 15 July 2010)
iForest Database Search
Search By Author
Search By Keyword
Google Scholar Search
Citing Articles
Search By Author
Search By Keywords
PubMed Search
Search By Author
Search By Keyword