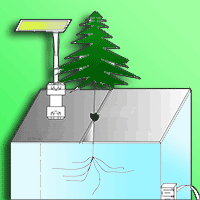
Relationship between volatile organic compounds released and growth of Cunninghamia lanceolata roots under low-phosphorus conditions
iForest - Biogeosciences and Forestry, Volume 11, Issue 6, Pages 713-720 (2018)
doi: https://doi.org/10.3832/ifor2797-011
Published: Nov 06, 2018 - Copyright © 2018 SISEF
Research Articles
Abstract
To understand whether Chinese fir (Cunninghamia lanceolata) can conserve energy by reducing root volatiles to maintain growth under low phosphorus (P) conditions, we cultivated two half-sib families of Chinese fir that display high and low P use efficiency under conditions of normal P supply and total P deficiency. Gas chromatography-mass spectrometry analysis was used to determine the content of root volatiles, and the relationships among root volatiles and root growth index, P content, and distribution were analyzed. There were significantly fewer volatile organic compounds (VOCs) in the rhizosphere of these two fir families, No. 25 and No. 32, under P deficiency. Low P supply significantly promoted root growth in No. 25, increasing both average diameter and volume. A negative correlation was found between the volatiles and the increment of root average diameter and surface area. The belowground P distribution and the root to shoot P concentration (Pr/Ps) were higher in No. 25 than in No. 32. The total amount of VOCs, as well as the amount of 18 individual volatiles were positively correlated with P accumulation, aboveground P distribution, and belowground P distribution, but the opposite pattern was seen in Pr/Ps for family No. 25 seedlings. We conclude that the content and types of VOCs differ among the Chinese fir genotypes. Under low-P stress, the roots of Chinese fir reduce the release of VOCs to maintain seedling growth.
Keywords
Cunninghamia lanceolata, Low-phosphorus Stress, Root Growth, Root Volatile Organic Compounds, Energy Balance
Introduction
Phosphorus (P) supports important functions in structural tissues and plays a crucial role in various metabolic processes in plants ([35]). However, available P in the soil is readily bound by calcium, iron, and aluminum, resulting in extremely limited amounts of P available to plants ([32]). In nature, some plants maximize P acquisition efficiency using mycorrhizal symbioses ([28]), rhizosphere modification through secretion of organic acids, protons, and phosphatases ([5], [27], [37]).
These adaptation mechanisms consume energy and undoubtedly increase the respiratory burden of root tissues ([22]). However, plants must balance income with investment ([22]). In order to maintain such a balance, some plants dissolve root cortical cells into the cavity, reducing respiratory needs and saving energy, and shift the dissolved P to meet the needs of other parts of the plant ([4], [34]). In some crop species, the root cortical aerenchyma are formed in the old root tissue to lower the respiratory consumption and ensure the normal growth of young tissues ([13]). Moreover, some plants or some genotypes within the same plant species effectively improve water and nutrient acquisition instead of accelerating root proliferation, as the metabolic costs of expanding the root system in the soil are very high ([22], [30]).
The release of volatile organic compounds (VOCs) produced by different plant organs (leaves, flowers, fruits, and roots) represents a loss of energy in the plants, but is known to be the key mediator in biotic interactions of both the shoot and root systems ([10], [16], [12], [26]). However, when plants coexist with individuals of the same species, they increase the secondary metabolism of phenols and antioxidants and reduce primary metabolism, such as leaf and root formation, in order to enhance their resistance to environmental stresses while maintaining a balance between energy generation and investment ([4]). In a study of Rosmarinus officinalis planted in a group, the phenolic compounds in leaves increased significantly, but the metabolism of lipids and amino acids decreased ([25]). In another study, bitou bush (Chrysanthemoides monilifera ssp. rotundata) released VOCs to inhibit the root growth of a neighboring plant (Isolepis nodosa [Rott.] R. Br) and maintain its dominance ([11]). Thus, adjusting the energy balance strategy is important for maintaining plant growth and coping with environmental stress ([21]).
In previous studies, Chinese fir (Cunninghamia lanceolata [Lamb.] Hook.), a unique fast-growing timber species in south China, has been reported to reduce its energy expenditure under low-P stress by significantly decreasing the leaf net photosynthetic rate, transpiration rate, and stomatal conductance ([6], [15]). However, the elite genotypes with high P use efficiency had a greater ability to forage available P patches, displaying increased root proliferation in the form of larger root surface area and root volume in P-poor than in P-rich patches ([31], [36]). How can this energy-consuming behavior be reconciled with the need to reduce energy expenditure under P deficiency? As VOCs are an important secondary metabolite, we hypothesize that: (1) under low P stress, Chinese fir decreases the release of VOCs in order to reduce energy expenditure, to different extents in different genotypes; and (2) the content of VOCs released is negatively correlated with plant growth traits, especially with root morphological traits.
In the present study, conducted in two Chinese fir half-sib families with different P use efficiencies, we measured the root growth increment, the average increase in root diameter, the root surface area increment, P accumulation, the P distribution pattern, and the release of VOCs from the rhizosphere of Chinese fir seedlings. The aim was to gain a better understanding of the energy metabolic balance associated with P acquisition in Chinese fir by studying the regulatory mechanism under low-P stress.
Materials and methods
Plant materials
Two half-sib Chinese fir families, No. 32 and No. 25, were selected from 1.5 generations of seed orchard at the state-owned forest farm in Zhangping, Fujian Province, SE China. These families were selected based on preliminary observations of significant differences (p < 0.05) in P utilization efficiency, with No. 32 showing lower efficiency than No. 25 under no-P conditions. For this preliminary experiment, six-month-old seedlings were planted in pots and harvested three months after the start of the treatments ([34]). Hence, we used healthy, six-month-old, uniform seedlings (14.02 ± 0.42 cm in height, 2.48 ± 0.10 mm in root collar diameter) from the forest farm as the test subjects. They were cultivated in washed sand containing available P at 0.034 ± 0.007 mg kg-1.
Experimental design
Each seedling was cultivated in ad-hoc designed acrylic pots, which were 15 cm long, 15 cm wide, and 20 cm in height (Fig. 1) in the greenhouse at the College of Forestry, Fujian Agriculture and Forestry University, starting on October 30, 2016. Temperature in the greenhouse ranged from 18 °C to 28 °C, and the relative humidity was 65.2% and 78.7% during the light and dark periods of the experiment, respectively. To each pot we added 4.5 kg of cleaned sand passed through a 2-mm sieve and 10 g crystal mud (Shenyang base stone, Shuanglong Chemical Co., China) that absorbed the Hoagland solution ([31]) for 24 h (1 g dry crystal mud, with a particle size of 1 mm, can absorb 100 mL solution).
Fig. 1 - Schematic presentation of the collection of gases from the roots of Chinese fir seedlings. The collection device was made of polyvinyl fluoride (PVF), which is chemically inert. The PVF sampling bag can store the gas over a long period of time with no change to the concentration. The test device was a square box with a top divided into two flaps, the left side leading to an air outlet that was connected to the collection bag outside the tube. On the right side, an open air inlet was fixed to an annular hose, with a uniform hole to ensure uniform flow of gas. A gas flow meter was connected to the air inlet, providing a flow of air through activated carbon cylinders to clean the air. VOCs released by the roots at the bottom of the tube flowed up to the air outlet. The sides of the tube, the bottom plate, and the top cover were 5 mm thick and made of acrylic material; the square pipe joints were watertight, and the entire test device was airtight when closed. To ensure that no air leaked out of the box, tape was used to seal the top flaps.
The seedlings from two families were assigned to one of two P levels, normal P (P1: 1.0 mmol L-1 KH2PO4) and no P (P0: 0 mmol L-1 KH2PO4). Each treatment was replicated five times. A 200-mL volume of deionized water was poured into the pot once every 2 days (at 7:00 p.m.), with water potential 70% (moisture probe mp-406, ICT International Pty, Armidale, NSW, Australia).
Data collection
VOC collection and detection
The rhizosphere VOCs were collected at 10:00 a.m. on day 7, 14, and 21 during the experiment. VOCs were collected three times from three replicates, as well as from two control containers that contained different P levels but no seedlings. The VOCs released in the pots were collected by dynamic headspace collection ([29]) as follows (Fig. 1). First, the left and right top flaps were closed. To fill any gaps between the planting trough and the stem of the seedling, a layer of cotton was wrapped around the stem of the seedling. Secondly, a 2-L volume of PVF (polyvinyl fluoride) sampling bag (model TD-4501, Dalian Deling Gas Packaging Co., Liaoning, China) was pumped to vacuum standby. A drum-type collection device was used with an air compressor to force the air through the activated carbon, pass the gas flow meter, through the sealed rhizosphere containing plants, and into the collection bag. Next, the air compressor was opened and the gas flow meter was adjusted to 20 L min-1, the system was ventilated for 2 minutes to drain the air from the unit, and the collection bag switch was opened. Finally, the collection bag switch and the air compressor switch were closed while the bag was filled. After collection, the sampling bag was wrapped in a black opaque plastic bag to prevent the VOCs from decomposing.
Roots can produce a large amount of VOCs such as terpenes ([19]). In the present study, 18 VOCs (Fig. 2) were screened and tested ([26]). The VOCs were analyzed by gas chromatography-mass spectrometry (GC-MS-QP7890B®, Agilent Technologies, Santa Clara, CA, USA) operating in EI mode at 70 ev, with a source temperature of 230°C, scanning range 35-350 amu, and sweeping speed at 3.125 u s-1. The GC-MS was fitted with a fused silica HP1 capillary column (60 m × 0.32 mm) with helium as the carrier gas with a flow rate of 4 mL min-1, and the injector temperature was set at 200 °C in splitless mode. The oven temperature program started at 10 °C and remained at that temperature for 3 min, increased by 5 °C min-1 until it reached 120 °C, then increased by 10 °C min-1 to 250 °C and remained at 250 °C for 7 min, with a 3-min scan. The compounds were identified by comparison with mass spectra in NIST 14.
Fig. 2 - The amounts and types of 18 volatile organic compounds (VOCs) released from Chinese fir roots after 21 days of low-phosphorus stress. Within each panel, bars (means ± SE) labeles by the same lowercase letter are not significantly different (p>0.05).
Root morphology traits and P distribution determination
The tested seedlings were harvested on November 20, 2016 (21 days after the treatments were initiated). All roots were carefully cleaned with distilled water and dried with filter paper, and the root system image was scanned. Then the root morphological traits such as root length, surface area, total volume, and average diameter were analyzed using WinRHIZO™ ver. 4.0B root analysis system software (Regent Instruments Inc., Sainte-foy, Quebec, Canada), after scanning by a Canadian Digital Scanner (STD1600®, Epson, USA). The increments in root morphological traits were calculated as differences in each trait before and after the treatments.
The harvested seedlings were divided into aboveground and belowground parts, oven dried at 105 °C for 30 min to deactivate enzymes, and then dried at 79 °C to constant mass to determine dry mass and P concentration. The aboveground and belowground parts of the seedlings were crushed, after which 0.2 g of the air-dried sample was passed through a 2-mm sieve and weighed. The crushed samples were digested with H2SO4-HClO4. The P concentrations of the seedlings were measured by phosphomolybdate following the method described in He et al. ([17]). We calculated aboveground P distribution by multiplying aboveground P concentration by aboveground dry biomass, and belowground P distribution following a similar process. The total P accumulation equaled aboveground P distribution plus belowground P distribution. To estimate the P translocation efficiency, we calculated root to shoot P concentration ratio (Pr/Ps).
Data analysis
The VOC components were analyzed by Masshunter Qualitative Analysis B.07.00 and Quantitative Analysis B.07.01 (Agilent Technologies, Santa Clara, CA, USA). Two-factors analysis of variance (ANOVA) was performed to determine the effects half-sib families (No. 25 and No. 32), P supply (P0 and P1) on the amount of VOCs released by Chinese fir roots, using the software package SPSS® ver. 19.0 (IBM, Armonk, NY, USA). In addition, the differences in growth, distribution of P, P accumulation per family, P supply, and the interaction of these factors were examined. Means that exhibited significant differences (p < 0.05) were examined by LSD multiple comparison method. Correlations between growth traits and P accumulation and between P distribution and VOC content at different P treatments were also analyzed, and differences were considered significant at the 0.05 level and highly significant at the 0.01 level (2-tailed). All data were expressed as mean ± standard error.
Results
VOC types and amounts released by roots of Chinese fir under P stress
There was significant two-way interaction effects between Chinese fir family and P supply level on the amounts of VOCs released by roots, and both factors significantly affected the VOCs amounts on an individual basis (p < 0.05 - Tab. 1).
Tab. 1 - Effects of Chinese fir family and phosphorus (P) supply level on root morphological traits, P allocation patterns and amount of volatile organic compounds (VOCs) released by roots of seedlings. F-values are given with their level of significance. (*): p < 0.05; (**): p < 0.01.
Group/Variable | Factors (F-values) | |||
---|---|---|---|---|
Family (F) |
P supply level (P) |
F × P | ||
Amount of VOCs | 7.40* | 34.48** | 9.32* | |
Increment of root morphology traits |
Root length | 68.22** | 0.37 | 1.47 |
Root surface area | 42.33** | 4.39 | 0.41 | |
Root average diameter | 18.33** | 13.43* | 5.63* | |
Root volume | 90.30** | 62.23** | 34.82** | |
P allocation patterns | P accumulation of whole plant | 3.32 | 14.97* | 0.005 |
P accumulation of root | 1.46 | 15.11** | 0.007 | |
P accumulation of shoot | 6.56* | 0.52 | 0.001 | |
Pr/Ps | 7.77* | 3.19 | 1.32 |
The amount of VOCs released by both Chinese fir families was lower under P deficiency (P0) treatment than under normal P supply (Fig. 3). As the duration of the experiment continued, the amount of VOCs decreased strongly and the difference in amount of VOCs reached a significant level under P deficient treatment, whereas the amount did not change significantly under normal P supply treatment. Interestingly, P deficiency promoted the release of 1-butene from family No. 32 seedling root at 21 days, while the amounts of other types of VOCs released from both two families were significantly less under P deficient than under normal P supply treatment (Fig. 2), similar pattern at 7 days and 14 days was observed (see Fig. S1 and Fig. S2 in Supplementary material for the original data used to perform this analysis).
Fig. 3 - Changes in the amounts of volatile organic compounds (VOCs) released by Chinese fir seedlings under different phosphorus supply conditions for different periods of time. Within each inflection point, bars (means ± SE) labeled by the same lowercase letter are not significantly different (p>0.05) in family no. 32 seedlings, by the same capital letter are not significantly different (p>0.05) in family no. 25 seedlings.
Relationship between root growth and amount of VOCs under P stress
Root growth
There was significant two-way interaction effects between Chinese fir family and P supply level on the increments of root average diameter and volume (p < 0.05), but not on the increments of root length and surface area (p > 0.05 - Tab. 1). Low P stress significantly promoted growth of average root diameter (Fig. 4A) and root volume (Fig. 4B) in family No. 25 seedlings compared with the family No. 32 seedlings. As individual factors, P supply level non-significantly affected either root length or surface area (p > 0.05). In contrast, the root morphology growth was significantly different in the two families (p < 0.05 - Tab. 1); both the increments of root length and surface area of family No. 25 seedling roots were larger than those of family No. 32 (Fig. 4C, Fig. 4D).
Fig. 4 - Difference in the root length increment (A), average root diameter increment (B), root surface area increment (C), and root volume increment (D) between two genotypes of Chinese fir seedlings (families no. 25 and no. 32) under low-phosphorus stress. Within each panel, bars (means ± SE) labeled with the same lowercase letter are not significantly different (p>0.05).
Correlation between root growth and amount of VOCs
For family No. 25 seedlings, the correlation between the amount of 18 individual VOCs and the increment of root average diameter was not significant (p > 0.05), but the increment of root volume was negatively correlated with the total amount of VOCs or the 18 individual VOCs (p < 0.05 - Tab. 2). For family No. 32 seedlings, there was a negative correlation between the amount of 16 individual VOCs and the increment of root average diameter, while the increment of root volume was positively correlated, the relationship with the release of 1-butene was inverse; however, neither of these measures reached a significant level (p > 0.05 - Tab. 2).
Tab. 2 - Relationship between root morphology traits and amount of volatile organic compounds (VOCs) released by roots of Chinese fir seedlings. (AD): Average diameter increment; (V): Volume increment; (L): Length increment; (SA): Surface area increment; (*): p<0.05 (2-tailed); (**): p< 0.01 (2-tailed).
VOC | Family no. 25 | Family no. 32 | ||||||
---|---|---|---|---|---|---|---|---|
AD | V | L | SA | AD | V | L | SA | |
Total VOCs | -0.79 | -0.94* | -0.65 | -0.24 | -0.58 | 0.06 | 0.02 | -0.62 |
Ethane | -0.8 | -0.93** | -0.68 | -0.17 | -0.56 | 0.05 | 0.02 | -0.6 |
Propane | -0.76 | -0.90* | -0.62 | -0.24 | -0.56 | 0.05 | 0.02 | -0.6 |
n-Butane | -0.72 | -0.96** | -0.71 | -0.26 | -0.41 | 0.13 | 0.04 | -0.11 |
Iso-butane | -0.59 | -0.84* | -0.90* | 0.002 | 0.65 | 0.51 | 0.7 | 0.78 |
2.3-Dimethylbutane | -0.79 | -0.93** | -0.65 | -0.24 | -0.53 | 0.08 | 0.02 | -0.6 |
Isopentane | -0.66 | -0.98** | -0.67 | -0.3 | -0.54 | 0.12 | 0.04 | -0.71 |
Cyclohexane | -0.74 | -0.94** | -0.7 | -0.3 | -0.49 | 0.04 | 0.08 | -0.82* |
3-Methylhexane | -0.8 | -0.94** | -0.68 | -0.19 | -0.5 | 0.08 | 0.01 | -0.57 |
n-Decane | -0.79 | -0.94** | -0.66 | -0.22 | -0.58 | 0.08 | 0.01 | -0.64 |
Ethene | -0.79 | -0.94** | -0.66 | -0.21 | -0.58 | 0.08 | 0.02 | -0.63 |
Propene | -0.75 | -0.94** | -0.59 | -0.35 | -0.51 | 0.1 | 0.03 | -0.63 |
1-Butene | -0.71 | -0.96** | -0.58 | -0.35 | 0.56 | -0.22 | 0.08 | 0.67 |
α-Pinene | -0.8 | -0.94** | -0.67 | -0.19 | -0.57 | 0.07 | 0.02 | -0.62 |
β-Pinene | -0.79 | -0.94** | -0.65 | -0.23 | -0.59 | 0.06 | 0.01 | -0.62 |
Styrene | -0.8 | -0.94** | -0.66 | -0.21 | -0.61 | 0.09 | -0.02 | -0.65 |
Benzene | -0.79 | -0.94** | -0.65 | -0.22 | -0.58 | 0.08 | 0.02 | -0.64 |
Methylbenzene | -0.76 | -0.93** | -0.57 | -0.36 | -0.6 | 0.02 | 0.01 | -0.63 |
m/p-Xylene | -0.77 | -0.91* | -0.52 | -0.32 | -0.56 | 0.11 | 0.02 | -0.67 |
Relation between P accumulation/allocation and VOC amount under P stress
P accumulation and allocation
The effect of Chinese fir family on P accumulation of root system was significant (p < 0.05 - Tab. 1). The P supply level had a significant effect both on P accumulation of the whole plant and the shoot (p < 0.05 - Tab. 1). Compared to P deficient treatment, the P accumulation of the whole plant and the shoot of families No. 25 and No. 32 were significantly lower than that of normal P supply treatment (p < 0.05 - Fig. 5A, Fig. 5B), but not in P accumulation of root system (p < 0.05 - Fig. 5C). Moreover, the P accumulation in the roots and the Pr/Ps were higher for family No. 25 than for family No. 32 seedlings (p < 0.05 - Fig. 5C, Fig. 5D).
Fig. 5 - Phosphorus (P) accumulation (A), aboveground P distribution (B), belowground P distribution (C), and ratio of belowground P concentration to aboveground P concentration (Pr/Ps - D) between two genotypes of Chinese fir seedlings (no. 25 and no. 32) under low-P stress. Within each panel, bars (means ± SE) labeled with the same lowercase letter are not significantly different (p>0.05).
Correlation between P accumulation and allocation with amount of VOCs
The total amount of VOCs and the 18 individual VOCs were positively correlated with P accumulation in the whole plant, shoots, and root system, but the opposite pattern was seen in Pr/Ps for family No. 25 Chinese fir seedlings (Tab. 3). Moreover, for family No. 25 seedlings, there was a significant correlation between P accumulation in the whole plant and the 18 types of volatiles except 1-butene (p < 0.05). For family No. 32, the correlation between the individual amount of 16 types of VOCs and P accumulation in the whole plant was positive, while Pr/Ps was positively correlated, the relationship with the release of either iso-butane or 1-butene being inverse; however, neither of these VOCs reached a significant level (p > 0.05 - Tab. 2).
Tab. 3 - Relationship between phosphorus allocation traits and amount of volatile organic compounds (VOCs) released by roots of Chinese fir seedlings. (PAW): P accumulation in whole plant; (APD): aboveground P distribution; (BPD): belowground P distribution; (Pr/Ps): root-shoot P concentration ratio; (*): p< 0.05 (2-tailed); (**): p< 0.01 (2-tailed).
VOC | Family no. 25 | Family no. 32 | ||||||
---|---|---|---|---|---|---|---|---|
PAW | APD | BPD | Pr/Ps | PAW | APD | BPD | Pr/Ps | |
Total VOCs | 0.88* | 0.71 | 0.19 | -0.49 | 0.73 | 0.86* | 0.09 | -0.76 |
Ethane | 0.91* | 0.66 | 0.16 | -0.53 | 0.75 | 0.86* | 0.06 | -0.76 |
Propane | 0.88* | 0.8 | 0.35 | -0.46 | 0.74 | 0.87* | 0.07 | -0.76 |
n-Butane | 0.86* | 0.73 | 0.3 | -0.53 | 0.8 | 0.78 | -0.13 | -0.5 |
Iso-butane | 0.84* | 0.59 | 0.5 | -0.59 | -0.39 | -0.19 | 0.2 | 0.09 |
2.3-Dimethylbutane | 0.89* | 0.68 | 0.15 | -0.48 | 0.76 | 0.84* | 0.05 | -0.76 |
Isopentane | 0.83* | 0.75 | 0.31 | -0.56 | 0.72 | 0.75 | 0.12 | -0.76 |
Cyclohexane | 0.84* | 0.77 | 0.32 | -0.44 | 0.68 | 0.7 | 0.1 | -0.8 |
3-Methylhexane | 0.90* | 0.68 | 0.18 | -0.52 | 0.77 | 0.85* | 0.03 | -0.76 |
n-Decane | 0.89* | 0.7 | 0.18 | -0.51 | 0.72 | 0.84* | 0.1 | -0.76 |
Ethene | 0.89* | 0.68 | 0.19 | -0.52 | 0.73 | 0.85* | 0.1 | -0.76 |
Propene | 0.82* | 0.76 | 0.21 | -0.43 | 0.77 | 0.79 | 0.05 | -0.76 |
1-Butene | 0.81 | 0.76 | 0.22 | -0.48 | -0.64 | -0.78 | -0.25 | 0.77 |
α-Pinene | 0.90* | 0.67 | 0.16 | -0.52 | 0.73 | 0.85* | 0.09 | -0.76 |
β-Pinene | 0.89* | 0.7 | 0.18 | -0.5 | 0.72 | 0.87* | 0.1 | -0.76 |
Styrene | 0.90* | 0.7 | 0.18 | -0.51 | 0.67 | 0.86* | 0.17 | -0.76 |
Benzene | 0.89* | 0.7 | 0.18 | -0.5 | 0.72 | 0.84* | 0.11 | -0.76 |
Methylbenzene | 0.83* | 0.82* | 0.28 | -0.41 | 0.69 | 0.90* | 0.1 | -0.77 |
m/p-Xylene | 0.85* | 0.82* | 0.27 | -0.44 | 0.72 | 0.79 | 0.12 | -0.76 |
Discussion
Different plant species produce different kinds of VOCs ([3]), and there are also notable differences between genotypes ([8]). The present study showed that the VOCs released by two Chinese fir families were different. Significantly higher amounts of iso-butane, cyclohexane, and ethane were released from family No. 25 seedlings in response to different P levels compared with family No. 32 seedlings, although more n-butane was released by family No. 32 seedlings. Under P deficiency, the total amounts of VOCs released by family No. 25 and 32 were lower than under normal P supply level (Fig. 3). With the increasing duration of P deficiency, the amounts of VOCs released was severely inhibited, and the difference in the amount of VOCs reached a significant level under P deficient condition. Evidently, the seedlings responded to P stress by reducing its secondary metabolism to conserve resources and shifting the limited remaining resources to more vital functions. More metabolic resources are required for tasks such as resource acquisition, growth, and reproduction ([20], [30]). However, P deficiency promoted the release of 1-butene from family No. 32 seedling root system in this study (Fig. 3); similarly, emission of a type of VOC (isoprene) increased in Phragmites australis exposed to low P concentrations ([14]). They found that isoprene emission was limited by processes other than the availability of photosynthetic byproducts or by energetic (ATP) requirements under high P supply levels.
The nutrient use efficiency of plants depends on resource availability and utilization. Chiou & Lin ([7]) reported that plants possess a multivariate signal network consisting of hormones, sugars, miRNA, and other modulators that work at different levels of regulation. The two Chinese fir families analyzed in this study (No. 25 and No. 32) used different strategies to cope with low-P stress, which may be the reason for the difference in the release of VOCs from their roots. Family No. 25, similar to other Chinese fir genotypes, exhibited “active capture of soil P,” and as such, mainly adapts to low-P conditions via strong root proliferation and exudation of chemical substances ([31], [36], [37]). In contrast, family No. 32 is like the “passive low-P tolerance” genotype and could produce a high yield when the available soil P concentration was lower than that required by the average genotype, which mainly resists low-P stress by accelerating the internal P cycle of the plant body, e.g., via improving the efficiency of P utilization by dissolving root cortex cells ([34]). In the present study, the root length and surface area of family No. 25 seedling were larger than those of family No. 32 (Fig. 4C, Fig. 4D). According to the principle of conservation of energy, genotypes with high P efficiency need to consume more energy ([13]) and to balance their energy metabolism by reducing the emission of root volatiles.
The metabolic cost of soil exploration is an important component of plant growth under low P availability ([21]). The lack of soluble P in the soil caused roots to expend more energy trying to detect P resources ([22]). The VOCs released by roots represent a metabolic cost, so reducing the VOCs is an adaptation to save energy ([2]). In the present study, VOCs produced by the root system of Chinese fir seedlings under P deficiency affected the P accumulation of the whole plant, the distribution pattern of P between the root and shoot portions of the seedlings, and root growth. As the duration of P stress was prolonged, significant differences developed in the amount of VOCs released by different families under different P treatments. Many principles associated with resource allocation and acquisition are analogous to optimization theory in economics, especially with regard to limited internal resources and constrained availability of external resources ([21]).
The genotype with high P use efficiency is well suited to economic analysis ([21]). Under P deficiency, the belowground P distribution and Pr/Ps of family No. 25 were higher than those of family No. 32. Increased relative allocation to root growth is obviously beneficial for P acquisition ([24]). The physiological responses to Chinese fir to no-P stress include altering the distribution and structure of roots ([31]), dissolution of root cortical cells ([4], [34]), catalytic hydrolysis of acid phosphatase ([5]), release of organic acids ([33]), and reduction of leaf net photosynthetic rate, transpiration rate, and stomatal conductance ([18]). It is of interest to determine whether the morphological and physiological changes occur in roots after the VOCs receive the signal molecules indicating the conditions.
Furthermore, VOCs released from the roots into the soil change the physical and chemical properties of the soil and affect the growth of adjacent plants ([1]). Plant roots can identify related species according to their VOC profile ([9]). For example, ethylene, a well-known phytohormone, is a critical component of the VOCs and can mediate the growth and P remobilization in the root systems by the expression of the P deficiency-responsive gene under low-P stress conditions ([23], [35]). The results of the present study show that under P deficiency stress, the root VOCs of Chinese fir influenced the accumulation of P and root morphology and were to some extent correlated. However, further research is needed to determine the other specific VOCs that promote physiological changes ([33]).
Conclusions
Under different P supply conditions, the type and quantity of root VOCs were significantly different in two Chinese fir families. Under total P deficiency, the amount of VOCs of family No. 25 and 32 was lower that than under P1 treatment. Low P supply significantly promoted growth of both average root diameter and root volume in family No. 25 seedlings compared with family No. 32 seedlings. For family No. 25, the increment of root volume was negatively correlated with 18 individual types or total amounts of VOCs. For family No. 32 seedlings, there was a negative correlation between 17 individual VOCs and the increment of root average diameter. Under P deficient treatment, the P accumulation of the whole plant and aboveground P distribution of family No. 25 and 32 seedlings were significantly lower than that of P supplied treatment. The types and amounts of 18 VOCs were positively correlated with accumulation of P, aboveground P distribution, and belowground P distribution, but the opposite pattern was observed for the Pr/Ps ratio in family No. 25 seedlings.
Acknowledgments
This research was financially supported by the National Natural Science Foundation of China (Grants no. U1405211, 31370531) and the Science and Technology Plan Project of Fuzhou City, China (2017-N-35). We would like to thank Professor Zhigang Yi and Xingran Huang and Wei Lin for help with our chemical analyses, Minghui Yang and Yuan Yang for help with the experiment, and Mulualem Tigabu for reviewing the article.
References
Authors’ Info
Authors’ Affiliation
Kai Wu
Nemin Wang
Wenjing Wu
Xianhua Zou
Xiangqing Ma
Pengfei Wu
College of Forestry, Fujian Agriculture and Forestry University, Fuzhou, 350002 (China)
Corresponding author
Paper Info
Citation
Lai H, Wu K, Wang N, Wu W, Zou X, Ma X, Wu P (2018). Relationship between volatile organic compounds released and growth of Cunninghamia lanceolata roots under low-phosphorus conditions. iForest 11: 713-720. - doi: 10.3832/ifor2797-011
Academic Editor
Claudia Cocozza
Paper history
Received: Mar 21, 2018
Accepted: Aug 21, 2018
First online: Nov 06, 2018
Publication Date: Dec 31, 2018
Publication Time: 2.57 months
Copyright Information
© SISEF - The Italian Society of Silviculture and Forest Ecology 2018
Open Access
This article is distributed under the terms of the Creative Commons Attribution-Non Commercial 4.0 International (https://creativecommons.org/licenses/by-nc/4.0/), which permits unrestricted use, distribution, and reproduction in any medium, provided you give appropriate credit to the original author(s) and the source, provide a link to the Creative Commons license, and indicate if changes were made.
Web Metrics
Breakdown by View Type
Article Usage
Total Article Views: 45757
(from publication date up to now)
Breakdown by View Type
HTML Page Views: 39819
Abstract Page Views: 2582
PDF Downloads: 2541
Citation/Reference Downloads: 3
XML Downloads: 812
Web Metrics
Days since publication: 2432
Overall contacts: 45757
Avg. contacts per week: 131.70
Article Citations
Article citations are based on data periodically collected from the Clarivate Web of Science web site
(last update: Mar 2025)
Total number of cites (since 2018): 4
Average cites per year: 0.50
Publication Metrics
by Dimensions ©
Articles citing this article
List of the papers citing this article based on CrossRef Cited-by.
Related Contents
iForest Similar Articles
Research Articles
Carbohydrate metabolism during new root growth in transplanted Larix olgensis seedlings: post-transplant response to nursery-applied inorganic fertilizer and organic amendment
vol. 10, pp. 15-22 (online: 22 September 2016)
Research Articles
Effects of nitrogen loading under low and high phosphorus conditions on above- and below-ground growth of hybrid larch F1 seedlings
vol. 11, pp. 32-40 (online: 09 January 2018)
Research Articles
Fine root morphological traits and production in coniferous- and deciduous-tree forests with drained and naturally wet nutrient-rich organic soils in hemiboreal Latvia
vol. 16, pp. 165-173 (online: 08 June 2023)
Research Articles
Effects of understory removal on root production, turnover and total belowground carbon allocation in Moso bamboo forests
vol. 9, pp. 187-194 (online: 20 November 2015)
Review Papers
Leaf volatile isoprenoids: an important defensive armament in forest tree species
vol. 5, pp. 13-17 (online: 14 February 2012)
Research Articles
Changes in organic compounds during leaf litter leaching: laboratory experiment on eight plant species of the Sudano-guinea Savannas of Ngaoundere, Cameroon
vol. 1, pp. 27-33 (online: 28 February 2008)
Research Articles
Modeling the fine root biomass dispersion using a special influence function
vol. 1, pp. 141-144 (online: 25 November 2008)
Research Articles
Fine root production and distribution in the tropical rainforests of south-western Cameroon: effects of soil type and selective logging
vol. 3, pp. 130-136 (online: 27 September 2010)
Research Articles
Links between phenology and ecophysiology in a European beech forest
vol. 8, pp. 438-447 (online: 15 December 2014)
Research Articles
Mechanical and physical properties of Cunninghamia lanceolata wood decayed by brown rot
vol. 12, pp. 317-322 (online: 06 June 2019)
iForest Database Search
Search By Author
Search By Keyword
Google Scholar Search
Citing Articles
Search By Author
Search By Keywords
PubMed Search
Search By Author
Search By Keyword