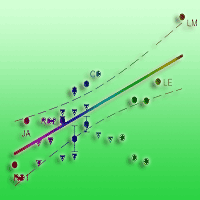
Soil C:N stoichiometry controls carbon sink partitioning between above-ground tree biomass and soil organic matter in high fertility forests
iForest - Biogeosciences and Forestry, Volume 8, Issue 2, Pages 195-206 (2015)
doi: https://doi.org/10.3832/ifor1196-008
Published: Aug 26, 2014 - Copyright © 2015 SISEF
Research Articles
Abstract
The release of organic compounds from roots is a key process influencing soil carbon (C) dynamics and nutrient availability in terrestrial ecosystems. Through this process, plants stimulate microbial activity and soil organic matter (SOM) mineralization thus releasing nitrogen (N) that sustains gross and net primary production (GPP and NPP, respectively). Root inputs also contribute to SOM formation. In this study, we quantified the annual net root-derived C input to soil (Net-Croot) across six high fertility forests using an in-growth core isotope technique. On the basis of Net-Croot, wood and coarse root biomass changes, and eddy covariance data, we quantified net belowground C sequestration. Belowground C accumulation and GPP were inversely related to soil C:N, but not to climate or stand age. Soil C content and C:N were also related to soil texture. At these high fertility sites, biomass growth did not change with soil C:N; however, biomass growth-to-GPP ratio significantly increased with increasing soil C:N. This was true for both our six forest sites and for another 23 high fertility sites selected at a global scale. We suggest that, at high fertility sites, plant N demand interacts with soil C:N stoichiometry and microbial activity, resulting in higher allocation of C to above ground tree biomass with increasing soil C:N ratio. When C:N is high, microbes have a low C use efficiency, respire more of the fresh C inputs by roots and prime SOM decomposition, thereby increasing N availability for tree uptake. Soil C sequestration would therefore decrease, whereas the extra N released during SOM decomposition can promote tree growth and ecosystem C sink allocation in aboveground biomass. Conversely, C is sequestered in soil when low soil C:N promotes microbial C use efficiency and new SOM formation and stabilization on clay particles.
Keywords
Net Root-derived Carbon, Ingrowth Cores, Soil C:N, Carbon Sequestration, Carbon Partitioning, Isotopes
Introduction
Forest ecosystems worldwide are currently acting as carbon (C) sinks ([70]). Several factors may, however, influence the magnitude and direction of the net C balance, including recovery from historical land use (e.g., abandoned agricultural land reverting to forested land), increases in atmospheric CO2 concentration and nitrogen (N) deposition, and climate change ([96], [108]). Nonetheless, while much research has been done to understand the controls on net ecosystem C balance ([111], [89], [85]), we know little about the controls on C sink partitioning between plant biomass and soil organic matter (SOM) pools. Soils may store C for long periods of time ([53]), accumulating on average three times the C in terrestrial vegetation ([76]). On the other hand, more N is required per unit of C stored in soil as compared to plant biomass ([118]). Hence, while an allocation to SOM may increase C sequestration in the long term, a preferential allocation to plant biomass is a more nutrient-efficient C sequestration process in the shorter term.
Studying ecosystem C sink partitioning is challenging due to the difficulties associated with quantifying the different ecosystem fluxes. Especially complex is the assessment of rapid and small changes in SOM which are linked to the balance between microbial respiration and plant inputs, including both litter and root-derived C ([98]). Thus, belowground C allocation and subsequent C dynamics are still far from being accurately quantified and understood ([74], [114]). Root C inputs have been shown to influence soil C sequestration, but both the magnitude and direction of this root effect are variable ([44], [72], [7], [82], [20]).
A robust definition of net ecosystem production (NEP
) should be based on a full ecosystem mass balance ([81]), which accounts for both plant and soil sinks. When it is flux-based, NEP
is defined as the difference between ecosystem-level gross photosynthetic gain of C (gross primary production, GPP
) and ecosystem respiratory losses (R
eco). Alternatively, NEP
(g C m-2 y-1) can be expressed as ([6] - eqn. 1):
In deciduous forest ecosystems, ΔC
biomass is the annual change in plant biomass (wood, branches, coarse roots), and ΔC
soil is the annual net change in soil organic C (SOC) stock. In this equation, litterfall and fine root turnover are considered as soil C input and therefore contributing to the ΔC
soil (see eqn. 2).
Net ecosystem productivity can be directly determined using eddy covariance techniques starting from net ecosystem exchange (NEE
= ’Â’NEP -
[3], [2]). Plant biomass changes are usually estimated via a combination of repeated inventories and allometric relationships ([10]). On the other hand, direct SOC determination methods are generally unable to quantify ΔC
soil in the short term ([98]), and, at annual timescales, alternative methods are required to estimate soil C changes.
Considering that the dissolved organic C (DOC) is typically negligible, representing around 1% of forest NPP
([59]), ΔC
soil can also be written as (eqn. 2):
where Input
litter is the above-ground litterfall (i.e., leaves, branches, wood, etc.), Input
roots is the root-derived C input (i.e., exudates, root slashing and turnover), R
C-rhizosphere is the rhizosphere respiration of root-derived C, and Rh
is the heterotrophic respiration. Litter input is conventionally measured by litter traps, while wood input is measured using repeated sampling ([34]), and rhizosphere and heterotrophic respiration can be estimated by a variety of methods (e.g., trenching, girdling, isotopes), as reviewed by Subke et al. ([104]) and Kuzyakov ([51]). The largest challenge is estimating gross root inputs. However, methods exist to estimate net annual root-derived C input (Net-C
root), which is the difference between Input
roots and R
C-rhizosphere (eqn. 3):
Different tracer methods have been used to date to estimate Net-C
root, such as pulse labeling, continuous labeling, and 13C natural abundance ([50]). The latter uses the difference in the stable C isotope composition of native SOM and new plant-derived organic matter to quantify Net-C
root.. When natural isotope abundances do not allow the use of this approach, distinct C isotope signatures in the soil organic C (SOC) pool and plant-derived organic matter can be obtained in manipulation experiments, by growing C3 plants (δ13C of approximately -27‰) in soil with organic matter derived from C4 plants (δ13C of approximately -12‰) or vice versa. This approach has been successfully applied in pot ([43], [113]) and field studies ([40], [12]) and was used in this investigation.
Net-C
root, combined with aboveground inputs to the soil (litter and dead wood), also provides interesting information about soil C dynamics. For soils at steady-state (ΔC
soil=0), the sum of Net-C
root and aboveground inputs is the amount of C that replaces SOC decomposition, thus becoming a measure for SOC turnover. For soils which are net C sinks (ΔC
soil>0), this sum exceeds SOC mineralization and a fraction of it enlarges the SOC pool, thus leading to soil C sequestration. In this context, for soils which are net C sinks, the ratio between ΔC
soil and Net-C
root + aboveground inputs indicates the fate of C input: the higher the ratio, the larger the contribution of fresh C to soil C sequestration. The opposite is true for soils that are net C sources (ΔC
soil<0).
Root C input rates vary considerably depending on tree species, mychorrhizal associations and environmental factors ([54]), with values of up to 40% of net assimilated C being reported ([112]). According to the microbial efficiency-mineral stabilization (MEMS) framework ([13]), the fraction of Net-C
root inputs sequestered in the soil depends on the efficiency of decomposers to convert C into bio-products as compared to tha amount of C lost as CO2 ([99]) and on soil matrix interactions ([100], [48]). Soil organic matter mineralization is driven by both substrate stoichiometry and microbial demand for resources ([67], [38]): when N is limiting, microbes use labile substrate to mineralize recalcitrant SOM ([69], [14]). Root exudates can thus prime SOM decomposition ([56], [5], [26]). Clearly, root-derived soil C inputs can either stimulate soil C sequestration or, conversely, induce priming with consequent losses of stabilized SOM but likely enhancements in N availability, which in turn can stimulate plant growth. The key factors determining the direction (and magnitude) of this effect are, however, not yet clear. Understanding the fate of root-derived C, and its effects on N dynamics and ecosystem C sequestration, is relevant from an ecological perspective and is also an urgent challenge to address, particularly in the context of global changes such as atmospheric CO2 increase and N deposition.
The aims of the present study were: (1) to obtain an estimate of Net-C
root in six different forest ecosystems; (2) to partition NEP into aboveground tree biomass production and soil C sinks; and (3) to investigate the controls of this partitioning. Specifically, we tested the hypothesis that soil C:N stoichiometry controls ecosystem C uptake (GPP
) and sink partitioning (ANPP
vs. soil C) across forest ecosystems. To verify if our hypothesis could be generalized to other forests, we tested it on several world forest sites for which ANPP
, GPP
and soil C:N data were available in the literature.
Materials and methods
Study sites
Six forests were considered in the present study. Three sites were in central Italy, two sites in northern Italy, and one in Croatia. All sites were equipped with an eddy covariance tower for mass, momentum and energy ecosystem exchange measurements and can be classified as high fertility sites, according to key soil properties ([114] - see also Appendix 1). Site characteristics and flux data are reported in Tab. 1, while a brief description for each site is given below.
Tab. 1 - General characteristics for the six forest sites used in this study. (ΔC
wood): change in aboveground wood biomass; (ΔC
roots): change in coarse root biomass; (NEP
): net ecosystem production; (NEE
): net ecosystem exchange; (GPP
): gross primary production; (R
eco): ecosystem respiration; (RO1): Roccarespampani site 1; (RO2): Roccarespampani site 2; (LE): Lecceto; (JA): Jastrebarsko; (LM): La Mandria; (CO): = Collelongo. (a): N wet deposition in 1990 were derived for all sites using published gridded maps with 0.5° × 0.5° resolution derived from interpolated (krieged) ground data (available at ⇒ http://www.daac.ornl.gov). Total wet depositions (kg N ha-1 y-1) were then computed as the sum of aqueous NO3- and NH4+ fields, which were available. (b): For Collelongo, the reported number refers to direct measurements available for the period 2002-2009 ([25]).
Group | Parameters | RO1 | RO2 | LE | JA | LM | CO |
---|---|---|---|---|---|---|---|
Vegetation characteristics | Main species | Turkey oak | Turkey oak | Holm oak | Pedunculate oak | Pedunculate oak - Hornbeam | Beech |
Management | coppice with standards | coppice with standards | coppice with standards | high forest | high forest | coppice converted to high forest since 1950 | |
Mean stand age in 2006-2007 (years) | 6 (approx. 70 standards ha-1 of 20-40 years-old present) |
15 (approx. 70 standards ha-1 of 20-40 years-old present) |
15 | 35 | 80 | 110 | |
Aboveground biomass (kg C m-2) |
1.9 | 4.5 | 5.0 | 6.3 | 7.8 | 13.7 | |
Wet N deposition1 (kg N ha-1 y-1) a |
10.0 | 10.0 | 8.6 | 11.2 | 9.4 | 10.8 b | |
Soil characteristics | Soil type | Volcanic Luvisol |
Volcanic Luvisol |
Xerocrept | Luvic Stagnosol |
Typic Fragiudalf |
Humic Alisols |
Bulk density (0-15 cm, g cm-3) |
1.29 | 1.29 | 1.20 | 0.77 | 1.09 | 0.58 | |
Bulk density (15-30 cm, g cm-3) |
1.25 | 1.25 | 1.20 | 1.11 | 1.27 | 0.66 | |
Soil C stock 0-30 cm (kg C m-2) | 8.6 | 7.4 | 27.4 | 7.7 | 7.4 | 14.7 | |
Soil N stock 0-30 cm (kg N m-2) | 0.8 | 0.5 | 1.3 | 0.7 | 0.3 | 0.9 | |
C:N | 10 | 14 | 22 | 11 | 24 | 16 | |
Sand (%) | 52 | 52 | 40 | 18 | 6 | 6 | |
Silt (%) | 12 | 12 | 35 | 28 | 80 | 50 | |
Clay (%) | 35 | 35 | 25 | 54 | 14 | 44 | |
Carbon fluxes | GPP (g C m-2 yr-1) |
1577 | 1356 | 901 | 1633 | 754 | 1258 |
R eco (g C m-2 yr-1) |
1060 | 810 | 368 | 1049 | 183 | 722 | |
ΔC wood (g C m-2 yr-1) |
161 | 315 | 334 | 325 | 360 | 363 | |
Root:shoot ratio | 0.30 ([68]) |
0.30 ([68]) |
0.30 ([68]) |
0.30 ([68]) |
0.30 ([68]) |
0.28 (assessed at the site) |
|
ΔC roots (g C m-2 yr-1) |
48 | 95 | 100 | 98 | 108 | 102 | |
ΔC biomass = ΔC wood +ΔC roots (g C m-2 yr-1) |
209 | 410 | 435 | 423 | 468 | 464 | |
Litterfall (g C m-2 yr-1) | 47 | 123 | 107 | 203 | 223 | 245 | |
NEP (= ’Â’NEE ) (g C m-2 yr-1) |
517 | 545 | 533 | 584 | 571 | 535 |
Roccarespampani (42° 24′ N, 11° 55′ E - [11], [107]) is a Turkey oak (Quercus cerris L.) coppice forest at about 235 m a.s.l. in central Italy. Mean annual temperature is 14 °C and mean annual rainfall is 755 mm. Soil is sandy clay Luvisol (which is typically nutrient rich), derived from sedimentary material of volcanic origin and marine deposits, and is moderately acid (pH=5.7), with a total depth > 100 cm ([87]). Cation exchange capacity (CEC) is high, ranging between 19 and 42 meq 100g-1 in the different soil layers ([107]). The forest has been managed as a “coppice with standards” over the last 200 years, with a rotation cycle varying between 15 and 20 years. Two stands were selected: a 6-year-old coppice (RO1) and a 15-year-old coppice (RO2).
Lecceto (LE - 43° 18′ N, 11° 16′ E) is a Holm oak (Quercus ilex L.) coppice with a rotation period of 18-20 years at about 300 m a.s.l. in central Italy. Holm oak represents 81% of the total tree canopy; others species include Arbutus unedo L., Juniperus communis L., Quercus pubescens L., Phillyrea latifolia L., Fraxinus ornus L. Mean annual temperature is 13.5 °C and annual average rainfall is 780 mm.
Jastrebarsko (JA - 45° 37′ N, 15° 41′ E; [64], [65]) is a 35-year-old forest in Croatia dominated by pedunculate oak (Quercus robur L.) with 19% of black alder (Alnus glutinosa Haernt.), 14% hornbeam (Carpinus betulus L.) and 9% of narrow-leafed ash (Fraxinus angustifolia L.). Mean annual temperature is 10.4 °C with mean monthly temperatures of -0.2 °C and 20.7 °C in January and July, respectively. Average annual precipitation is 900 mm year-1, of which around 500 mm falls during the active vegetation period (April-September). Soil is a Luvic Stagnosol with a depth > 100 cm and an acidic pH (4.9) in the upper mineral layer (0-20 cm) that linearly increases to neutral pH at depths > 100 cm. At the beginning of the growing season, the soil drains and water content soon drops below water holding capacity (46% v/v) allowing enough oxygen supply for root growth and substantially increasing nutrient availability in these soils, where nutrient availability can be constrained by high water levels.
La Mandria (LM - 45° 09′ N, 7° 34′ E) is an 80-year-old pedunculate oak-hornbeam forest (Quercus robur L. and Carpinus betulus L.) in northern Italy. Mean annual temperature at the site is 11.6 °C and annual precipitation is 1030 mm. Soil is Typic Fragiudalf with adequate moisture content throughout the year, neutral pH and good CEC (ranging from 17 to 11 meq 100 g-1 at soil surface and Bh horizons, respectively).
Collelongo (CO - 41° 52′ N, 13° 38′ E; [110], [95]) is an 110-years-old pure beech (Fagus sylvatica L.) forest in northern Italy that has been part of the network of Long Term Ecological Research sites (LTER Italy) since 2006. Mean annual temperature at the site is 7.1 °C and mean annual rainfall is 1188 mm. The soil is a Humic Alisol with volcanic ash also present. Both CEC and N content are high in the different soil layers, ranging from 14.8 to 23.3 meq 100g-1 and from 4 to 7.3 mg N g-1, respectively ([73]). Wet N deposition rates in the period 2002-2009 averaged 10.8 kg N ha-1 yr-1 ([25])
Net root-derived C input to soil
Net-C
root was quantified using the in-growth core isotope technique, following Cotrufo et al. ([12]). A soil depleted in 13C (δ13C = -17.22‰) was collected from the USDA-ARS Central Plains Experimental Range located in NE Colorado, USA (40° 49′ N, 104° 46′ W). The soil is classified as a Zigweid soil series (Fine-loamy, mixed, superactive, mesic Ustic Haplocambid), with a pH of 7.4, N content of 1.37 g kg-1, and P content of 0.5 g kg-1 ([12]). At this site, plant cover is approximately 75% C4 grasses, and for brevity we call henceforth this soil as “C4 soil”. Soil was air-dried prior to being sealed and boxed for shipment to Italy. Upon arrival, the C4 soil was ground and sieved to 2 mm and well mixed to make a homogeneous soil pool, before using it for in-growth cores and chemical (C% and δ13C) analyses as described below.
At each forest site, six cores, made of a 2 mm mesh net (thus allowing the penetration of fine roots) with a diameter of 4 cm and a height of 30 cm, were placed randomly within the eddy covariance tower footprint in October 2006 (2008 for Jastrebarsko) and filled with the C4 soil to a bulk density similar to the average bulk density for the site. At the top of each core the net was closed to avoid above-ground litter input. Cores were sampled a year later, and the soil from each core was separated into 0-15 cm and 15-30 cm depth layers, except for Jastrebarsko, where the entire 0-30 cm core was considered.
All soil samples were sieved to 2 mm, and root samples carefully removed and washed with deionized water. Root samples were pooled by site and depth, and each samples analyzed in triplicates. Both soil and root samples were oven-dried at 70 °C, pulverized and analyzed for %C and δ13C by an elemental analyzer (Flash EA 1112 NC, CE Instrument, Wingan, UK) connected to an Isotope Ratio Mass Spectrometer (IRMS, Delta Plus, Thermo-Finnigan, Bremen, Germany). Prior to C analyses, soil samples were treated with HCl to eliminate carbonates ([36]). The measured δ13C values were used to calculate the proportion of new C (f
new, i.e., the Net-C
root), by using a mass balance equation ([17], [12] - eqn. 4):
where δ
soil is δ13C of the organic matter of the C4 soil collected from each core after one year of field incubation, δ
old is the δ13C of the organic matter of the C4 soil measured before incubation, and δ
veg is the δ13C of the roots averaged by site and depth. The average δ
veg value across all our sites was -28.11±0.29‰, while variation (standard deviation) within a site was between 0.15 and 0.57‰ at RO1 and RO2, respectively. Knowing the f values for the new C, the soil organic C concentrations (%C), soil depth (D, m), and soil bulk density (σ, kg m-3), Net-C
root amounts (g m-2) were computed for all soil samples as follows (eqn. 5):
Estimates of Net-C
root using this method ([12]) rely on the assumptions that: (1) root inputs are the same inside and outside the in-growth bags and are independent of the C4 soil properties; and (2) that there is no isotopic fractionation during the decomposition of the native SOM or formation of the new SOM from the root tissues. New studies applying this method should test those assumptions, since some fractionation could occur ([39]).
Ecosystem fluxes and primary production
Eddy covariance flux data from all five Italian sites were analyzed for the years 2006-2007 (Tab. 1). Data of net ecosystem exchange (NEE
), gross primary production (GPP
) and ecosystem respiration (R
eco) at monthly time steps were downloaded from the central Fluxnet database (⇒ http://gaia.agra⇒ ria.unitus.it/database/). Specifically, we used the NEE gap-filled data using the Artificial Neural Network method (NEE_ANN from level 4 dataset - [71]). R
eco was computed according to the short-term temperature response of night-time fluxes ([84]) and GPP
values were derived as sum of the absolute values of NEE_ANN and R
eco. At sites where data for the years 2006 or 2007 were incomplete even after gap-filling because of missing weather data, data for 2008 were also included in the analysis for the calculation of annual means. As for the Jastrebarsko site, 2009 eddy flux data were derived from Marjanovic et al. ([64]).
Mean annual temperature (MAT), mean annual precipitation (MAP), and soil C stocks (0-30 cm), as well as changes in wood biomass (stem and branches - ΔC
wood), were derived from ancillary data files available at the central database, updated to 2006-2007 when necessary, or using specific yield tables available at the site (e.g., Jastrebarsko). All data were checked, if necessary updated and completed by site Principal Investigators, who are co-authors of the present study. Changes in root biomass (ΔC
roots) were derived from ΔC
wood using root-to-shoot ratios reported by Mokany et al. ([68]) or using site-specific relationships as in the case of Collelongo and do not include fine root productivity.
ANPP
was calculated as the sum between ΔC
biomass and NPP
leaves (foliar net primary production). The latter corresponds to litterfall in the case of broadleaved forests, and was directly measured at the site (i.e., Rocca, Jastrebarsko, Collelongo) or assessed from NPP
wood using biomass expansion factors derived at nearby sites with similar species composition and structure (i.e., La Mandria). In the case of Lecceto, where the dominant species is evergreen (Holm oak), we assumed that the system was at steady state and thus litterfall = NPP
leaves. Then the ANPP
: GPP
ratio was calculated.
World forest sites data
In order to test if the relationship between ANPP
:GPP
and soil C:N, observed across our study sites, was generalizable across forest ecosystems, we searched published datasets ([55], [58], [114]) for forest sites that provided the data suitable to our analyses. Twenty-three additional sites were found including ANPP
and GPP
data, as well as soil C:N (determined for a depth up to 45 cm) were found (Tab. 2). Fertility classification followed Vicca et al. ([114]). More details are given in Appendix 1.
Tab. 2 - Studies used to validate the relationship between ANPP
:GPP
and soil C:N across gradients of forest stands and environmental conditions. Nutrient availability was assessed according to Vicca et al. ([114]), when possible. (n.a.): not available. (1): Ryan et al. ([92]), Binkley et al. ([4]); (2) Gholz et al. ([30]), Gholz & Fisher ([28]), Gholz et al. ([29]); (3) Gower et al. ([31]), Ryan et al. ([91]), Online BOREAS dataset. Site: “NSA-OJP-9OJP1”; (4): Malhi et al. ([60]), Marland et al. ([63]); (5) Malhi et al. ([60]), Luizao et al. ([57]); (6): Chambers et al. ([8]), Luizao et al. ([57]); (7) Harris et al. ([35]), Marland et al. ([63]); (8): Kinerson et al. ([46]); (9): Woodwell & Botkin ([117]); ANPP
= NPP
/1.3; Marland et al. ([63]); (10): Kutsch et al. ([49]); (11) Dilly et al. ([19]); (12): Sun et al. ([105]); (13): Malhi et al. ([61]); (14): Kelliher et al. ([45]); (15) Kelliher et al. ([45]); (16): Malhi et al. ([61]); (17): Present study; ANPP
= ΔC
wood + litterfall.
Forest type and location | Management or treatment |
Nutrient availability |
ANPP
|
GPP
|
ANPP : GPP |
Depth (cm) |
Soil C:N |
---|---|---|---|---|---|---|---|
Eucalyptus saligna plantation, Pepeekeo, HI1 |
2 yr, 1x1 m | high | 1427 | 5057 | 0.28 | 0-45 | 16 |
6 yr, 1x1 m | high | 480 | 2369 | 0.20 | 0-45 | 15 | |
2 yr, 3x3 m | high | 1456 | 4413 | 0.33 | 0-45 | 16 | |
6 yr, 3x3 m | high | 828 | 2930 | 0.28 | 0-45 | 15 | |
Pinus radiata plantation, Canberra, Australia1 |
20 yr C | medium | 599 | 2415 | 0.25 | 0-30 | 19 |
Pinus elliotii plantation, Bradford, FL2 |
7-9 yr | low | 199 | 1407 | 0.14 | 0-15 | 12 |
Picea mariana, N-BOREAS3 | 150 yr | low | 132 | 563 | 0.23 | n.a. | 13 |
Pinus banksiana, N-BOREAS3 | 63 yr | low | 115 | 677 | 0.17 | n.a. | 15 |
Oak-Hickory, Oak Ridge, TN4 | 55 yr | low | 510 | 1329 | 0.38 | 0-20 | 16 |
Tropical forest, Manaus Brazil5 | Old growth | low | 870 | 2620 | 0.33 | 0-10 | 14 |
Tropical forest, Manaus Brazil6 | Old growth terra firme | low | 650 | 2860 | 0.23 | 0-10 | 12 |
Liriodendron, Oak Ridge, TN7 | 50 yr | n.a. | 352 | 2162 | 0.16 | 0-20 | 14 |
Pinus taeda, Oak Ridge, TN8 | 16 yr | n.a. | 1490 | 4124 | 0.36 | 0-20 | 15 |
Pinus-Quercus, Oak Ridge, TN9 | 43 yr | n.a. | 462 | 1280 | 0.36 | 0-20 | 14 |
Bornhoved Alder10 | Temperate Humid-Broadleaved | low | 589 | 2420 | 0.24 | 0-30 | 18 |
Bornhoved Beech11 | Temperate Humid-Broadleaved | medium | 601 | 1324 | 0.45 | 0-5 | 15 |
Cascade Head (1)12 | Temperate Humid-Needle-leaved | high | 569 | 1400 | 0.41 | 0-30 | 21 |
Cascade Head (1A)12 | Temperate Humid-Broadleaved | high | 640 | 1558 | 0.41 | 0-30 | 20 |
Caxiuana13 | Tropical Humid-Broadleaved | low | 869 | 3630 | 0.24 | 0-30 | 13 |
Jacaranda/K3413 | Tropical Humid-Broadleaved | low | 796 | 3040 | 0.26 | 0-30 | 17 |
Metolius14 | Temperate Semi-arid-Needle-leaved | medium | 183 | 1143 | 0.16 | 0-30 | 20 |
Metolius young15 | Temperate Semi-arid-Needle-leaved | medium | 104 | 724 | 0.14 | 0-30 | 21 |
Tapajos 6716 | Tropical Humid-Broadleaved | low | 1400 | 3141 | 0.45 | 0-30 | 15 |
Rocca 117 | Meditteranian Turkey oak | high | 208 | 1577 | 0.13 | 0-30 | 10 |
Rocca 217 | Meditteranian Turkey oak | high | 438 | 1356 | 0.32 | 0-30 | 14 |
Lecceto17 | Meditteranian Holm oak | high | 441 | 901 | 0.49 | 0-30 | 22 |
Jastrebarsko17 | Meditteranian Pedunculate oak | high | 528 | 1633 | 0.32 | 0-30 | 11 |
La Mandria17 | Meditteranian Pedunculate oak - Hornbeam | high | 583 | 754 | 0.77 | 0-30 | 24 |
Collelongo17 | Meditteranian mountain beech | high | 608 | 1258 | 0.48 | 0-30 | 16 |
Data analysis
At each site the annual change in net soil C (ΔC
soil - g C m-2 y-1) was calculated starting from eddy covariance NEE
data and measured changes in aboveground wood biomass (ΔC
wood) and coarse roots (ΔC
roots) by re-arranging eqn. 1 (eqn. 6):
Statistical analyses were performed using the package SIGMA PLOT® 11.0 (Systat® Software, San José, CA, USA). Data were tested for normal distributions, using the Shapiro-Wilk’s test, and homogeneity of variance, and log transformed when necessary. To assess differences in Net-C
root among sites, a one way analysis of variance (one-way ANOVA) was used. Significant treatment (site) effects (P<0.05) were further explored via a treatment (site) comparison using the Least-Squares means test with Tukey’s adjustment for multiple comparisons. For sites where data for 0-15 and 15-30 cm depths were available, a two-way ANOVA with site and depth as fixed factors was also performed.
A correlation analysis between all available variables was performed using the Spearman’s rank method through a correlation matrix in Stata10® (StataCorp®, College Station, TX, USA). For variables that were correlated with p<0.10, linear models were fitted to measured data.
Results
Net root-derived C input to soil
Total Net-C
root in the top 30 cm soil layer ranged between 420 g C m-2 year-1 at Collelongo and 818 g C m-2 year-1 at Jastrebarko (Fig. 1). Mean annual Net-C
root across sites was 606 ± 164 g C m-2 year-1 (mean ± standard deviation). A significant difference in total Net-C
root (0-30 cm) was detected among sites (ANOVA, p = 0.007). In particular, post-hoc Tukey’s tests showed a significant difference between Jastrebarsko and Collelongo (p = 0.013) and Lecceto and Collelongo (p = 0.041 - Fig. 1). For sites where data for 0-15 and 15-30 cm depths were available (i.e., all sites except Jastrebarsko), the two-way ANOVA applied showed significant differences among sites (p = 0.004), between depths (p = 0.024) and for site × depth interaction (p = 0.035). No differences among sites were detected at 0-15 cm depth (Tukey’s test: p > 0.05), while Net-C
root at 15-30 cm in Lecceto was significantly different from Rocca1 (p = 0.002), Collelongo (p = 0.003) and Rocca2 (p = 0.021). Climate (i.e., MAT, MAP, soil water content) did not explain significant variability in Net-C
root among the different sites (see Tab. S1 in Appendix 1). Moreover, total Net-C
root was not significantly correlated to soil C:N.
Fig. 1 - Net annual root-derived carbon input (Net-C
root) to soils (0-15 and 15-30 cm) quantified using isotope-labelled (e.g., C4) soil in-growth cores at the six study sites. Vertical bars indicate standard deviation. Different letters indicate significant difference in total Net-C
root at p < 0.05. For site labels, see Tab. 1. As for the Jastrebarsko site (JA), only total net derived carbon is reported (grey bar).
Ecoystem C sink partitioning
All six sites were net C sinks with similar NEP
values (average NEP was 547 ± 25 g C m-2 year-1) but with large differences in annual GPP
(Tab. 1). They actively sequestered C both aboveground and in the soil: ΔC
wood represented between 10 and 48% of annual GPP
(RO1 and LM, respectively), ANPP
(=ΔC
wood + litterfall) was between 13 and 77% (RO1 and LM, respectively) and ΔC
soil was positive for all sites representing between 6 and 20% of annual GPP (CO and RO1, respectively).
In accordance with our hypothesis, GPP
and ΔC
soil were correlated to soil C:N (p = 0.0048 and p = 0.07, respectively - Tab. S1 in Appendix 1) and decreased linearly as soil C:N increased (Fig. 2). Moreover, GPP
was linearly and inversely related to soil clay content (p = 0.05) but, similarly to ΔC
soil, it was not correlated with either MAT, MAP or stand age. ΔC
wood was weakly related with soil C:N (p = 0.07), while ΔC
wood-to-GPP
ratio (Fig. 3.a) and ANPP
-to-GPP
ratio significantly increased with soil C:N (p < 0.0001 and p = 0.005, respectively - Tab. S1 in Appendix 1). In contrast to GPP
, Spearman’s correlation analysis revealed a significant relationship between ΔC
wood and both MAT and MAP (p = 0.05 and p =0.008, respectively - Tab. S1 in Appendix 1). Similarly, ANPP
was significantly correlated with MAT and MAP (p = 0.001 and p = 0.0003, respectively). Finally, the fate of root C input appeared to depend on soil C:N stoichiometry, with proportionally more Net-C
root being allocated to C sequestration with decreasing soil C:N (ΔC
soil vs. Net-C
root p = 0.07) and soil C:N was able to explain 40% of the variation in the ratio of ΔC
soil to Net-C
root + litterfall (Fig. 3.b).
Fig. 2 - Relationships between soil C:N stoichiometry and gross primary productivity (GPP
- panel A) or net soil C sequestration (panel B) as related to soil C:N ratio. Dashed lines represent 95% confidence interval; the reported R2 is the adjusted R2.
Fig. 3 - Relationships between soil C:N stoichiometry and ΔC
wood-to-GPP
ratio (panel A), or ΔC
soil : (Net-C
root + litterfall) ratio (panel B). Dashed lines represent 95% confidence interval; the reported R2 is the adjusted R2.
World forest sites
The positive relationship between ANPP
-to-GPP
ratio and soil C:N found across our six study sites was confirmed also when including additional fertile forests from different regions (Tab. 2). In particular, a positive relationship was found between ANPP
:GPP
and soil C:N for high fertility (sensu [114]) sites (adjusted-R2 = 0.64; p = 0.03; Fig. 4). Conversely, no significant relationship was detected for low and medium fertility sites.
Fig. 4 - Relationships between soil C:N stoichiometry and the ANPP
:GPP
ratio at forest sites with different fertility levels. For the complete list of sites see Tab. 2. Dashed lines represent 95% confidence intervals. The six sites considered in the present study are labeled as reported in Tab. 1. Eucalyptus saligna plantations (Tab. 2) have been averaged by stand age and vertical bars indicate standard deviation. The reported R2 is the adjusted R2.
Discussion and conclusions
To our knowledge, this study is the first to quantify Net-C
root in a range of forest ecosystems. The measurement of Net-C
root in situ is difficult, thus measured values are lacking and modeled estimates cannot be validated. However, the in-growth core isotope technique has already been shown to allow detection of changes in Net-C
root in CO2 and climate manipulation experiments ([40], [12]), even though it does suffer from several caveats related to the use of an exogenous soil and high spatial variability. Steingrobe et al. ([103]) reviewed the in-growth core method for measuring gross root growth: a first shortcoming associated with this method is achieving the soil conditions inside the bag similar to the bulk soil. Moreover, soil texture has also been shown to significantly influence rhizodeposition rates ([94]), although it is difficult to determine whether soil texture influenced rhizodeposition rates in our study.
Our estimates of Net-C
root using the in-growth core isotope technique were on average 606 g C m-2 y-1, which is higher than values reported by Cotrufo et al. ([12]) for a Arbutus unedo L. coppice in dry Mediterranean conditions, but lower than values reported by Hoosbeek et al. ([40]) for an irrigated and fertilized poplar plantation in central Italy. A possible overestimation of Net-C
root can be also related to the fact that a certain amount of fine root fragments could have passed through the 2 mm sieve. Such an amount is a function of root integrity as affected by plant age and sample processing. Being aware of this possible overestimation and of the above-mentioned limitations associated with the in-growth core isotope technique, in this study we used Net-C
root estimates solely as an indicator of differences in the effect of root-derived C on SOC sequestration through the calculation of the ratio ΔC
soil : (Net root-derived C + litterfall C).
Many factors have been suggested to affect soil C sequestration, including the characteristics of input material, soil texture and mineralogy, climatic factors, and soil nutrient status ([27], [1], [52]). We found that the proportion of root C input resulting in C sequestration at these high fertility sites was related to soil C:N ratio, and soil C sequestration was greater at low C:N (Fig. 2b) therefore confirming our hypothesis. Recently, Manzoni et al. ([62]) suggested a C-to-nutrient stoichiometric control on microbial C use efficiency (CUE), which would increase with increasing nutrient availability. The importance of CUE as a determinant of the fate of plant inputs to soils has also been recognized by other recent studies ([97], [13]) and some models have suggested that low nutrient availability, particularly N, might limit soil C storage through mechanisms that are still not completely understood ([83], [41]). Recently, Kirkby et al. ([47]) hypothesized that the sequestration of C-rich crop residue material into SOM could be improved only by adding supplementary nutrients, as the more stable SOM fraction has more N, P and S per unit of C than the plant material input due to microbial reprocessing. Thus, the increase in soil C sequestration at lower soil C:N values observed in this study may be explained by a higher microbial CUE of root C inputs.
Soil C:N exerted a strong control on GPP
across our six forests and GPP
increased with decreasing soil C:N (Fig. 2a). This relationship is based on six forest sites and we cannot exclude the possibility that other factors influenced this relation. At the ecosystem scale, variation in global plant productivity across ecosystems has often been related to environmental factors ([24], [86]), but also to nutrient availability ([114]). In this context, Zha et al. ([120]) reported a strong positive relationship between GPP
or NPP
and total soil N. Across our sites, ΔC
wood and ANPP
increased slightly, but not significantly with increasing soil C:N, and showed significant relations with MAT, MAP and stand age, thus confirming previous studies ([15], [42], [88], [37]). How such different behavior between GPP
and ΔC
wood or ANPP
with respect to soil C:N could be explained? We suggest that this result is due to the lower demand for N by woody tissues (which comprise the largest fraction of the tree and are characterized by very high C:N) as compared to green leaves (which control GPP
, and have much lower C:N than woody tissues). As a result of these variations in both GPP
and ANPP
, the ratio between ANPP
and GPP
varied substantially among our six forest sites.
Following the distinct patterns of ANPP
and GPP
versus soil C:N, the ANPP
-to-GPP
ratio significantly increased with increasing soil C:N (Fig. 2a). At first sight, this seems to contradict the current understanding that partitioning of photosynthates into aboveground biomass increases with increasing nutrient availability across a wide range of forests ([114]). However, all six forest sites had high nutrient availability but, at those sites where soil N presumably exceeded tree demand for wood growth (i.e., sites with low C:N), root C inputs were probably responsible for the higher net soil C sequestration. We speculate that the link between soil C:N stoichiometry and microbial activity controls C sequestration belowground, as well as for the increase in ANPP
-to-GPP
ratio with increasing soil C:N across the high fertility forests in our dataset. At soil C:N below 15, CUE is expected to be high, and more of the fresh C input is used for microbial products, resulting in the net formation of new SOM. Conversely, when C:N is high, microbes have a low C use efficiency and therefore they respire more of the fresh C inputs and prime SOM decomposition ([26]), which increases N availability and supports a higher allocation of fixed C (GPP
) to ANPP
. Our observations of increasing ANPP
-to-GPP
ratio, and the tendency for a decrease in soil C sequestration with increasing soil C:N (Fig. 2b), support this hypothesis.
In order to further test this hypothesis, we analyzed a larger dataset. Also in this case, ANPP
-to-GPP
ratios were quite variable (average ANPP
:GPP
= 0.28 with SD = 0.10; Tab. 2) and our analysis confirmed the relationship between ANPP
-to-GPP
ratio and soil C:N at sites with high fertility (Fig. 4). At sites where overall nutrient availability was low, this relationship did not hold. Variation in partitioning of GPP
to ANPP
at these sites is probably driven by the need for plants to invest in the nutrient acquiring system (i.e., roots and root symbionts - [114]). When nutrient availability is limited, belowground input by plants may be the dominant control of microbial activity and SOM mineralization ([33], [116], [16], [18]), thereby influencing mineral nutrient availability for plant uptake. Our speculation is also consistent with other recent findings. At the Duke Free Air CO2 Enrichment (FACE) experiment, the increase in the belowground C flux stimulated microbial activity, accelerated SOM decomposition, and stimulated tree uptake of N bound to this SOM, sustaining ANPP ([21], [22], [75]). Yin et al. ([119]) found that an increase in the release of root exudates into the soil was an important physiological mechanism to sustain growth responses of plants to experimental warming.
At our study sites, soil C:N stoichiometry appeared to be weakly controlled by the soil clay content (p = 0.15 - Tab. S1 in Appendix 1), decreasing with increasing %clay in soil. This is consistent with our knowledge of soil primary organo-mineral particles, which describes clay-associated SOM as the fraction with the highest microbial contribution and lowest C:N ratio ([9], [32]).
In conclusion, our results suggest that a specific site property, such as soil texture, could drive soil C:N stoichiometry which in turn would control ecosystem C uptake and partitioning within forests of high nutrient availability. While GPP
strongly and linearly increased with increasing soil N, aboveground tree biomass demand for N appeared to saturate, possibly because of the higher C:N of wood vs. green leaves, and, at high nutrient availability, NPP
became limited by other environmental factors. When this occurs, more C is sequestered by soil (Fig. 5), where the high N availability promotes CUE efficiency and new SOM formation.
Fig. 5 - Schematic representation of the proposed dynamics of gross (GPP
) and aboveground net primary production (ANPP
) with changes in nutrient availability. At high fertility sites (such as the sites considered in the present paper), GPP
, ANPP
and soil C sequestration (ΔC
) changes are controlled by soil C:N stoichiometry. At low soil C:N ratio, C sink allocation shifts from NPP
to soil C sequestration.
Acknowledgements
This work was financially supported by the Italian government through the FIRB project “CarboItaly”. We thank all site investigators, their funding agencies, the various regional flux networks (Afriflux, AmeriFlux, AsiaFlux, CarboAfrica, CarboEurope-IP, CarboExtreme, ChinaFlux, Fluxnet-Canada, KoFlux, LBA, NECC, OzFlux, TCOS-Siberia, USCCC), development of measurement and data submission protocols (funded by Office of Science (BER), US Department of Energy), and the Fluxnet project, whose work and support was essential for obtaining the measurements without which the type of integrated analyses conducted in this study would have not been possible. SV is a postdoctoral research associate of the Fund for Scientific Research - Flanders. Collelongo is a research site of the Italian network of Long Term Ecological Research (LTER-Italy).
References
CrossRef | Gscholar
CrossRef | Gscholar
CrossRef | Gscholar
Gscholar
CrossRef | Gscholar
Gscholar
CrossRef | Gscholar
CrossRef | Gscholar
CrossRef | Gscholar
Gscholar
Gscholar
CrossRef | Gscholar
CrossRef | Gscholar
Gscholar
Gscholar
CrossRef | Gscholar
Gscholar
CrossRef | Gscholar
CrossRef | Gscholar
CrossRef | Gscholar
Gscholar
CrossRef | Gscholar
Gscholar
Gscholar
CrossRef | Gscholar
Gscholar
Gscholar
CrossRef | Gscholar
Authors’ Info
Authors’ Affiliation
Giorgio Matteucci
Alessandro Peressotti
Department of Agriculture and Environmental Sciences, University of Udine, Udine (Italy)
Research Group of Plant and Vegetation Ecology, Department of Biology, University of Antwerp, Universiteitsplein 1, B-2610 Wilrijk (Belgium)
Department of Environmental Science, Second University of Naples, Caserta (Italy)
Department for Innovation in Biological, Agro-food and Forest systems, University of Tuscia, Viterbo (Italy)
Earth and Climate Cluster, Department of Earth Sciences, VU University Amsterdam (The Netherlands)
Franco Miglietta
Cristina Martinez
Institute of Biometeorology, National Research Council of Italy - CNR/IBIMET, Firenze (Italy)
Franco Miglietta
MOUNTFOR Project Centre, European Forest Institute, Research and Innovation Centre, Fondazione Edmund Mach (FEM), v. E. Mach 1, I-38010 San Michele all’Adige, TN (Italy)
Croatian Forest Research Institute, Jastrebarsko (Croatia)
Foxlab Joint CNR-FEM Initiative, v. E. Mach 1, I-38010 San Michele all’Adige, TN (Italy)
Institute for Agriculture and Forestry System in the Mediterranean, National Research Council of Italy - CNR/ISAFOM, Rende, CS (Italy)
Ettore D’Andrea
Institute of Agroenvironmental and Forest Biology, National Research Council of Italy - CNR/IBAF, Monterotondo, RM (Italy)
Istituto per le Piante da Legno e l’Ambiente - IPLA, Turin (Italy)
Department of Sustainable Agro-ecosystems and Bioresources, Research and Innovation Centre, Fondazione Edmund Mach (FEM), v. E. Mach 1, I-38010 San Michele all’Adige (Italy)
Department of Soil and Crop Science, Colorado State University, Fort Collins, Colorado (USA)
Corresponding author
Paper Info
Citation
Alberti G, Vicca S, Inglima I, Belelli-Marchesini L, Genesio L, Miglietta F, Marjanovic H, Martinez C, Matteucci G, D’Andrea E, Peressotti A, Petrella F, Rodeghiero M, Cotrufo MF (2015). Soil C:N stoichiometry controls carbon sink partitioning between above-ground tree biomass and soil organic matter in high fertility forests. iForest 8: 195-206. - doi: 10.3832/ifor1196-008
Academic Editor
Giustino Tonon
Paper history
Received: Dec 06, 2013
Accepted: Jul 13, 2014
First online: Aug 26, 2014
Publication Date: Apr 01, 2015
Publication Time: 1.47 months
Copyright Information
© SISEF - The Italian Society of Silviculture and Forest Ecology 2015
Open Access
This article is distributed under the terms of the Creative Commons Attribution-Non Commercial 4.0 International (https://creativecommons.org/licenses/by-nc/4.0/), which permits unrestricted use, distribution, and reproduction in any medium, provided you give appropriate credit to the original author(s) and the source, provide a link to the Creative Commons license, and indicate if changes were made.
Web Metrics
Breakdown by View Type
Article Usage
Total Article Views: 64363
(from publication date up to now)
Breakdown by View Type
HTML Page Views: 51766
Abstract Page Views: 5222
PDF Downloads: 5931
Citation/Reference Downloads: 56
XML Downloads: 1388
Web Metrics
Days since publication: 3961
Overall contacts: 64363
Avg. contacts per week: 113.74
Article Citations
Article citations are based on data periodically collected from the Clarivate Web of Science web site
(last update: Mar 2025)
Total number of cites (since 2015): 37
Average cites per year: 3.36
Publication Metrics
by Dimensions ©
Articles citing this article
List of the papers citing this article based on CrossRef Cited-by.
Related Contents
iForest Similar Articles
Review Papers
Separating soil respiration components with stable isotopes: natural abundance and labelling approaches
vol. 3, pp. 92-94 (online: 15 July 2010)
Research Articles
Potential relationships of selected abiotic variables, chemical elements and stand characteristics with soil organic carbon in spruce and beech stands
vol. 14, pp. 320-328 (online: 09 July 2021)
Research Articles
Soil stoichiometry modulates effects of shrub encroachment on soil carbon concentration and stock in a subalpine grassland
vol. 13, pp. 65-72 (online: 07 February 2020)
Research Articles
Effects of tree species, stand age and land-use change on soil carbon and nitrogen stock rates in northwestern Turkey
vol. 9, pp. 165-170 (online: 18 June 2015)
Research Articles
Voluntary carbon credits from improved forest management: policy guidelines and case study
vol. 11, pp. 1-10 (online: 09 January 2018)
Research Articles
Modeling air pollutant removal, carbon storage, and CO2 sequestration potential of urban forests in Scotlandville, Louisiana, USA
vol. 9, pp. 860-867 (online: 22 September 2016)
Research Articles
Estimating biomass and carbon sequestration of plantations around industrial areas using very high resolution stereo satellite imagery
vol. 12, pp. 533-541 (online: 12 December 2019)
Review Papers
Comparative assessment for biogenic carbon accounting methods in carbon footprint of products: a review study for construction materials based on forest products
vol. 10, pp. 815-823 (online: 25 September 2017)
Research Articles
Forest management with carbon scenarios in the central region of Mexico
vol. 14, pp. 413-420 (online: 15 September 2021)
Research Articles
Effects of understory removal on root production, turnover and total belowground carbon allocation in Moso bamboo forests
vol. 9, pp. 187-194 (online: 20 November 2015)
iForest Database Search
Search By Author
- G Alberti
- S Vicca
- I Inglima
- L Belelli-Marchesini
- L Genesio
- F Miglietta
- H Marjanovic
- C Martinez
- G Matteucci
- E D’Andrea
- A Peressotti
- F Petrella
- M Rodeghiero
- MF Cotrufo
Search By Keyword
Google Scholar Search
Citing Articles
Search By Author
- G Alberti
- S Vicca
- I Inglima
- L Belelli-Marchesini
- L Genesio
- F Miglietta
- H Marjanovic
- C Martinez
- G Matteucci
- E D’Andrea
- A Peressotti
- F Petrella
- M Rodeghiero
- MF Cotrufo
Search By Keywords