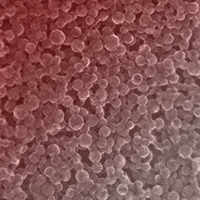
Can forest trees take up and transport nanoplastics?
iForest - Biogeosciences and Forestry, Volume 15, Issue 2, Pages 128-132 (2022)
doi: https://doi.org/10.3832/ifor4021-015
Published: Apr 09, 2022 - Copyright © 2022 SISEF
Research Articles
Abstract
Plastic contamination of ecosystems has increased dramatically over the last decades, raising concerns about the negative impacts of plastic particles on aquatic and terrestrial systems. In recent years, the focus of most research has shifted from large fragments (macroplastic) to micro- (<5 mm) and more recently to nano-plastic (<1000 nm) particles as more evidence has come to light about their ubiquity in water, soils, and living systems, and their effects on ecosystem and human health. In this study, we investigate nanoplastic uptake in the roots of seedlings (1-2 years old) of three different tree species and assess their transport to different tissues. Parts of the main roots of silver birch (Betula pendula Roth), sessile oak (Quercus petraea Matt. [Liebl.]), and Norway spruce (Picea abies [L.] Karst.) were immersed for one or four days in a suspension containing 13C-labelled nano-sized polystyrene particles (13C-nPS; 99% 13C, d = 28 ± 8 (1 σ) nm). Carbon stable isotope analysis showed significant 13C enrichment (P < 0.05) in the immersed part of the root after one day of treatment in all three species, and after four days in Q. petraea alone. Signals of significant 13C enrichment were also found in the aboveground tissues of the trees. The stem of B. pendula in particular showed a significant 13C enrichment after one day of treatment (P < 0.01). This indicates that nanoplastic particles can be taken up through tree roots into the tree’s central cylinder, where they are subsequently conveyed through the tree by acropetal transport via the xylem.
Keywords
Introduction
Plastics are synthetic polymers derived mainly from petroleum. Plastic production rates have increased steadily over the past decades, as have the attendant rates of waste production and pollution ([19], [16]). A lightweight, low-cost product, plastic is also resilient, durable, and easily transported and is therefore ubiquitous in modern life. The longevity of plastic is also the reason for its accumulation in the environment. Plastics have become a source of pollution affecting almost every ecosystem on the planet.
Plastic pollution is currently a key concern for human society, and its mitigation is a big challenge for future research and policy making ([27]). Plastic litter starts as macroplastics, such as bottles or packaging, which slowly fragment into micro- (<5 mm) and nano-sized particles (<1000 nm - [1]). Such small particles can rapidly disperse across many ecosystems ([11]). Most studies to date have focused on aquatic systems, such as rivers, lakes, and oceans. However, only about 5% of the annual terrestrial plastic waste ends up in marine ecosystems. The fate of the remaining plastic litter is still largely unknown due to the fragmentation of plastic into nanoparticles ([19], [10]).
Research has only recently started to focus on terrestrial ecosystems after decades of scrutinizing the fate and impact of plastics on marine and freshwater ecosystems. Microplastics have been found in floodplain soils ([32]), agricultural soils ([30]), forests ([9]), and glaciers ([3]). The range of ecosystems in which these particles are found indicates that micro- and nanoplastics can be transported by wind ([29]), and are therefore likely to also contaminate forest ecosystems. Atmospheric transport seems to be the most important pathway explaining the presence of plastic in remote areas and regions worldwide ([13], [14], [5], [7], [8], [26]). However, the fate of micro- and nanoplastics in the different ecosystems is almost unknown due to the analytical challenge of their detection in the environment ([35], [20], [28]).
A current challenge is to understand the micro- and nano-sized plastic pools and fluxes in terrestrial ecosystems, as well as the impact of plastic particles on plants and ecosystem functioning. Microplastics can affect the biophysical properties of soil, but our understanding of the complex relationships between microplastics, soil abiotic properties, microbial communities, and plants is still limited ([11], [24], [25]). Wang et al. ([36]) recently found that microplastics affect physical, chemical, and microbiological soil properties and that polymer type, dose, shape, and size can have different impacts in soils. Changes in soil properties may then affect the growth and development of plant roots, with potential consequences for ecosystem functioning.
Microplastics and nanoplastics can be absorbed by plant root hairs ([4]). Indeed, Bosker et al. ([6]) found a germination delay effect following the accumulation of microplastics in the root hairs of cress seedlings. Sun et al. ([33]) recently demonstrated nanoplastic uptake in Arabidopsis thaliana (L.) Heynh. by root tips and subsequent negative physiological effects. Giorgetti et al. ([17]) showed that onion seeds germinating in polystyrene nanoplastic suspensions exhibited decreased root growth and signs of cyto- and genotoxicity. In contrast, experiments with aquatic macrophytes have shown that growth depression only occurs when nanoplastic concentrations in the sediment exceed concentrations unlikely to be found in the environment ([34]). Van Weert et al. ([34]) used solutions with 0.03, 0.1, 0.3, 1, and 3% nanopolystyrene concentrations, which covers the range of concentrations likely to be found in the environment. An experiment with duckweed by Dovidat et al. ([12]) showed that although nanoplastic particles attached to roots, they were not detected within the plant. Li et al. ([22]) demonstrated submicrometer plastic uptake in crop plants via a crack-entry pathway through roots. In a recent hydroponic experiment, Liu et al. ([23]) found evidence of both nano- and micro-plastics uptake in rice seedlings through the roots and subsequent transport to aerial parts. Apoplastic transport was assumed to be the main pathway for plastic particles reaching aboveground tissues. Nanoplastic absorption by roots from colloidal solutions and transport in higher plants (Murraya exotica L.) has been shown by Zhang et al. ([38]). As with this study, the authors found that transport did not occur in the xylem and instead assumed it to be restricted to the apoplast of the lignified epidermis of roots and stems.
At present, there is a limited understanding of the impact of nanoplastics on tree physiology and forest health, and it is still unclear whether trees are able to take up nanoplastic particles via their roots. To assess whether and, if so, to what extent nanoplastics are taken up by trees through their roots, we immersed the roots of seedlings from three different forest tree species in a 13C-labelled nano-sized polystyrene particle suspension (13C-nPS) with a concentration similar to that observed in soils of polluted terrestrial ecosystems ([18], [37]). We further investigated whether nanoplastics can be transported to different aboveground tissues.
Materials and methods
Nanoplastic preparation, pre-processing, and characterization
Styrene-13C8 (Sigma-Aldrich, Buchs, Switzerland, ≥99 atom % 13C) was used to synthesize batches of spherical 13C-nPS of 28±8 (1 σ) nm in size following the procedure of Al-Sid-Cheikh et al. ([2]). Unreacted monomers were removed by ultrafiltration (exclusion size of membrane: 30.000 g mol-1). The hydrodynamic diameter (z average) of the particles was determined by dynamic light scattering (DLS) on a Zetasizer (NanoZS®, Malvern Instruments, UK). Secondary electron images recorded on a dedicated scanning transmission electron microscope (STEM, HD 2700 Cs®, Hitachi, Japan) indicated that the particles were mostly spherical and not aggregated (Fig. S1 in Supplementary material).
Plant material and greenhouse setting
In February 2019, 36 seedlings of three different tree species, previously grown outdoors in a forest nursery, were potted into 12 cm diameter plastic pots with a mixed soil substrate (“Containererde”, Ökohum GmbH, Switzerland). One-year-old silver birch (Betula pendula Roth), two-year-old sessile oak (Quercus petraea Matt. [Liebl.]), and two-year-old Norway spruce (Picea abies [L.] Karst.) were used. As each plant was potted, its main root was directed through a central hole at the bottom of the pot so that it was protruding out of the pot. This part of the root was then put into a smaller pot containing the same substrate, beneath the first pot (a sketch of the experiment set-up is shown in Fig. 1a).
Fig. 1 - Experiment set-up (not to scale). Average stem heights of the different forest tree species were: 18 cm for Q. petraea, 20 cm for P. abies, and 42 cm for B. pendula. (a) Illustration of the potting preparation of a forest seedling; (b) illustration of a seedling during the experimental phase. The five different plant tissues that were analysed are also indicated (R1, R2, R3, S, L).
This set-up enabled easy access to part of the rooting system for the 13C-labelling procedure (see below). The seedlings were then grown under natural light conditions in a greenhouse. The position of each seedling in the greenhouse was randomly changed once a week, and the pots were watered to field capacity twice a week.
Nanoplastic uptake experiment
At the end of August 2019, the main root of each seedling was carefully removed from the lower pot and rinsed with demineralised water to remove any adhering soil particles. The tips of the main roots (R1) were inserted into 15 ml Falcon® tubes (VWR, Dietikon, Switzerland) containing 12 ml of quarter-strength Hoagland nutrient solution (Fig. 1b). On the same day, 13C-nPS were added to the nutrient solutions of six plants of each species to reach a 0.2% mass concentration (according to [18]). The other six individuals of each species were used as controls. The acropetal part of the main root (R2) that was not immersed in the suspension (Fig. 1b) was covered daily with a fresh wet paper towel to prevent desiccation during the experiment.
For each species and treatment, three seedlings were sampled after one day of exposure to the 13C-nPS. The remaining three seedlings were sampled four days after the 13C-nPS was added. For the four-day-long exposure, the amount of suspension in the tubes was monitored daily and nutrient solution was added as needed to account for evaporation and absorption by the roots.
Plant harvest
After one or four days of treatment, the tips and the rest of the main roots that were immersed in the 13C-nPS suspension, plus a section of the root that was moistened by the suspension (~ 1cm, following [15]), were collected from each plant (R1 - Fig. 1b). The individual root pieces were washed intensively with demineralised water for five minutes, then dried with a paper towel and weighed using a precision laboratory balance (PM 200, Mettler-Toledo, Columbus, OH, USA). The rest of the plant was subdivided into the following sections (see Fig. 1b): the upper part of the main root outside of the exposure medium (R2), the remaining part of the root system in the main pot (R3), the stem (S), and the leaves (L). The roots (R3) were washed with tap water to remove adhering soil particles. All sections were weighed to obtain fresh weights, then oven dried at 60 °C for four days to obtain dry weights.
Stable isotope analysis
After drying, each of the five different tissues of the seedlings were homogenized using a ball mill for 1.5 minute at a frequency of 30 cycles per second (MM 400®, Retsch, Haan, Germany). For every tissue, first controls and then treatments were milled to avoid any possible contamination. One milligram of the homogenised material was weighed into a tin capsule then combusted in an elemental analyser (EA-1110®, Carlo Erba, Milan, Italy). The resulting CO2 was analysed in a coupled isotope-ratio mass-spectrometer (Delta Plus XL®, Thermo, Bremen, Germany). The ratio of 13C/12C in the sample indicates its relative deviation in per mil from the international standard, V-PDB, which is given as δ13C. Laboratory standards and international standards with known δ13C values were used for the calibration of the measurements, resulting in a precision of 0.2‰ for δ13C.
Data analysis and statistics
For each of the different tree species, we calculated the difference in δ13C between the tissues of the plants treated with 13C-nPS and the control plants. We refer to this difference in δ13C as Δδ13C. Positive Δδ13C values indicate 13C-enrichment in treated plants. We used RStudio Team 2020 to test for the significance of 13C enrichment by performing a mixed analysis of variance (ANOVA), an analysis of variance (ANOVA), and a one-sided t-test for each of the five tissues. These tests detect 13C-incorporation in or adsorption to a specific tissue in a specific species and at a specific time. Finally, the 13C mass balance for each tissue in each plant was calculated to quantify the amount of 13C or 13C excess (in g 13C) contained in each tree’s compartment (Tab. S1 in Supplementary material).
Results and discussion
The addition of 13C-labelled nanopolystyrene led to a very high enrichment in the incubated parts of the roots (Fig. 2). It also caused a significant overall δ13C increase in all three tree species, indicating that trees were able to take up nanopolystyrene through their roots and incorporate it in their tissues (ANOVA - Tab. 1).
Fig. 2 - Differences in 13C between plants with 13C-nanopolystyrene (Δδ13C) and controls. The Δδ13C between the plants treated with 13C-labelled nanopolystyrene and the control plants for various tissues of all three tree species at different times of exposure (1d= one-day treatment; 4d= four-day treatment). Means and standard errors of 3 replicates. Black stars indicate statistically significant differences (P < 0.05) between control and treatment, tested by a one-sided t-test. (R1): lower part of the main root, which was immersed in the nutrient solution; (R2): upper part of the main root, which was not immersed in the nutrient solution but was in contact with the air during the experiment; (R3): remaining part of the root system that was in the soil of the large pot.
Tab. 1 - ANOVA testing the effects of exposing three tree species to 13C-labelled nanopolystyrene for one and four days on δ13C values in various tissues. F-values from ANOVA are shown. (***): p< 0.001; (**): p< 0.01; (*): p< 0.05.
Factor | df | All tissues |
Root1 (R1) |
Root2 (R2) |
Root3 (R3) |
Stem | Leaves |
---|---|---|---|---|---|---|---|
Tissue | 4 | 66.8*** | - | - | - | - | - |
Treat | 1 | 74.8*** | 178*** | 8.14** | 0.05 | 2 | 4.63* |
Species | 2 | 4.1* | 0.79 | 0.69 | 7.8** | 6.9** | 17.9*** |
Time | 1 | 8.4** | 22.7 | 0.02 | 1.3 | 0.58 | 0.01 |
Species × Treat | 2 | 0.6 | 0.2 | 0.82 | 2.15 | 40.3*** | 1.41 |
Tissue × Treat | 4 | 49.2*** | - | - | - | - | - |
The part of the rooting system immersed in the polystyrene solution (R1) showed positive Δδ13C values in all three species after both one- and four-day-long treatments (P < 0.0001), indicating 13C enrichment (Fig. 2). The 13C enrichment is statistically significant (one-sided t-test: P < 0.05) in all three species following the one-day treatment, and in Quercus petraea following the four-day treatment (Fig. 2). Studies of both freshwater plants and an ornamental shrub have shown that nanoplastics can attach to the root surfaces ([38], [12]). If the intensive root washing failed to remove all 13C-nPS particles, the enrichment could reflect strong binding to the root surfaces and/or particle uptake via the roots ([33]).
The part of the root not immersed in the polystyrene solution (R2) showed positive Δδ13C values in all three species (P < 0.01, Tab. 1) and hence 13C enrichment. Seven out of 18 labelled seedlings showed Δδ13C values > 5‰, which is well above the natural variability of δ13C in unlabelled seedlings, with a standard deviation of 0.8‰.
In the part of the root system that remained in the soil (R3), the labelling did not change δ13C values significantly. However, the Δδ13C R3 value of one out of 18 treated P. abies seedlings (after one day of treatment) exceeded the standard deviation of control trees, indicating a 13C enrichment (Fig. 2).
Leaf tissues showed significant differences in Δδ13C at the treatment level (P < 0.05 - Tab. 1). A slightly positive Δδ13C value was found in B. pendula after one day of treatment (0.14 ‰ ± 0.47 - Fig. 2), but this value was not statistically significant.
In stem tissues, Δδ13C values depended upon tree species (treat × species: P < 0.001 - Tab. 1). The enrichment of δ13C in the stems of P. abies and Q. petraea remained below detection limit, but the stem tissues of B. pendula (Δδ13C = 2.28 ‰ ± 0.45) were significantly enriched (one-sided t-test: P < 0.01) after one day of treatment (Fig. 2), indicating the presence of nanopolystyrene. Estimating the 13C excess according to Ruehr et al. ([31]) revealed that this enrichment of B. pendula stems represents 0.19% of the total 13C-labelled polystyrene added to the exposure media (Tab. S1 in Supplementary material). This percentage corresponds to the ratio between the average 13C content in the stem tissue (4.44 · 10-5 g) and the average 13C content in the incubation solution (0.0232 g). In details, the incubation solution contained 0.0232 g of 13C (12.6 ml, or approximately 12.6 g) solution with a polystyrene concentration of 0.2%. This results in 0.0252 g of polystyrene per incubation vial, with approx. 93% of the molecules consisting of C and 99% labeled with 13C. Thus, the 13C content in the incubation vial is 0.0232 g 13C. Whereas the average 13C content in the stem tissue (4.44 · 10-5 g) is the result of the following multiplication: 2.495 · 10-5 (13C atom% excess equalling the enrichment of 2.28 ‰) · 3.56 g stem tissue biomass (g dry weight) · 0.5 (C content in g C per g dry weight).
The enrichment in the stem may be the result of 13C uptake in the central cylinder of the root and subsequent acropetal transport via the xylem. An alternative explanation, as suggested by Zhang et al. ([38]), is that 13C-nPS is transported in the apoplast of the lignified root epidermis without crossing the endodermis (and thus without reaching the central cylinder).
The 13C enrichment in the stem of B. pendula (Fig. 2) and the overall treatment effect for leaves (Tab. 1) suggests that long-distance transport of nanoplastics from the roots to the shoot occurs in trees. Betula pendula is an early successional species with high water use ([21]); its transpiration rates are higher than those of the two late successional species studied here (Q. petraea and P. abies). Xylem transport rates might therefore explain the significant accumulation of labelled 13C in the stems of B. pendula but not in the stems of the other two species.
It is important to note that the 13C signal of added nanopolystyrene gets diluted in seedling biomass when transported out of the treated part of the root into the other plant compartments. For example, if one assumes that the majority of the 5% of the label found in the R1 root of P. abies after one day of treatment (Δδ13C = 1237‰ ± 19 - Fig. 2) is uniformly transported to R2, R3, and aboveground tissues, this label is diluted by a factor of 0.021 (the biomass of R1 is 2.1% of the biomass of R2 + R3 + S + L; see Tab. S1 in Supplementary material). This would result in an average 13C enrichment of 1.30‰. The 13C enrichment in the stems of B. pendula exceeds this value (Δδ13C = 2.28‰ ± 0.45), implying higher uptake of nanoplastics. The 13C enrichment in the root parts R2 and R3 and stems of P. abies after one day of treatment is in this range but is not significant due to the high variability among individuals.
Even if signs of 13C enrichment were found in all the tissues, Δδ13C values of the aboveground tissues were close to the detection limit, revealing some discrepancies between the two different exposure times. When comparing the two exposure times, more statistically significant values were found after one day of treatment as compared after four days of treatment. We speculate that nanoparticles change root morphology and functioning over time, resulting in reduced uptake and transport to other tissues. Indeed, Zhang et al. ([38]) found that polystyrene nanoparticles created a physical barrier in the root micropores and significant injuries at the epidermal root cell level. We therefore assume that longer incubation times did not lead to higher uptake in our experiment. This does not explain the lower enrichment after 4 days of exposure (found in some cases), but this may be due to the fact that 13C enrichment was mostly close to the detection limit.
In conclusion, the use of 13C-nPS in our experiment gave some first evidence of the potential uptake of nanoplastics in trees. The highest 13C enrichments from 13C-nPS were obtained from roots immersed in the exposure media and may be the result of particle adsorption on the root surface. We speculate that 13C-nPS enters into roots via a crack-entry mode, as described by Li et al. ([22]). 13C-nPS might be transported to the stem tissue in some species (B. pendula) via the transpiration stream in the xylem. Although the 13C enrichment in stems of B. pendula was significant and an overall treatment effect on leaves was observed, 13C enrichment remained low, which can most likely be attributed to dilution in the large stem biomass. Future experiments with different exposure times, higher concentrations of 13C-nPS, or the use of more easily detected isotopes (e.g., 14C) would help to identify the magnitude of within-tree transport of nanoplastics. As indicated by this study, the uptake of nanoplastics by trees may affect tree physiological functions and allow nanoplastics to enter the food chain in forest ecosystems, as has been observed in marine environments.
Acknowledgements
We would like to express our thanks to Brian Sinnet (EAWAG) for his advice and help running the DLS analyses and to our colleagues at the WSL Claudio Cattaneo and Gabor Reiss for helping in the greenhouse; Dr. Jobin Joseph, Dr. Leonie Schönbeck, Shengnan Ouyang, and Dr. Marco Pecchia for helping to set up the experiment and collect data; Liska Dällenbach, Luc Schnell, Nadja-Tamara Studer, and Dr. Nasrullah Khan for helping with the harvest; and Manuela Oettli for running the isotope-ratio mass-spectrometer analysis. We would like to thank Ms. Erin Gleeson of SciencEdit.CH for her help revising a previous draft of this paper. We acknowledge financial support from the Swiss Federal Research Institute WSL.
Authors’ contributions
Conceptualization: MEM, PC, AG, IB, FH; Methodology: MEM, PC, AG, IB, FH, RG, MASC; Collection of data: MEM, PC, AG, PB; Data analysis: MEM, AG, MS, FH; Writing-original draft preparation: MEM, PC, AG, IB, FH; Writing-review and editing: MEM, AG, PC, IB, RG, MS, PB, FH, MASC, GO; Funding acquisition: PC, AG; Supervision: PC, AG, IB, FH.
References
CrossRef | Gscholar
CrossRef | Gscholar
CrossRef | Gscholar
CrossRef | Gscholar
Authors’ Info
Authors’ Affiliation
Paolo Cherubini 0000-0002-9809-250X
Ivano Brunner 0000-0003-3436-995X
Matthias Saurer 0000-0002-3954-3534
Paula Ballikaya 0000-0003-3044-3462
Frank Hagedorn 0000-0001-5218-7776
Arthur Gessler 0000-0002-1910-9589
WSL Swiss Federal Research Institute, Birmensdorf (Switzerland)
Department of Forest and Conservation Sciences, Faculty of Forestry, University of British Columbia, Vancouver BC (Canada)
Eawag, Swiss Federal Institute of Aquatic Science and Technology, Dübendorf (Switzerland)
Department of Chemistry, University of Surrey, Stag Hill, Guildford, GU2 7XH, Surrey (United Kingdom)
Research Platform “Data”, Leibniz Centre for Agricultural Landscape Research (ZALF), Müncheberg (Germany)
Berlin-Brandenburg Institute of Advanced Biodiversity Research (BBIB), Berlin (Germany)
Institute of Terrestrial Ecosystems, ETH Zurich, Zurich (Switzerland)
Corresponding author
Paper Info
Citation
Murazzi ME, Cherubini P, Brunner I, Kägi R, Saurer M, Ballikaya P, Hagedorn F, Al Sid Cheikh M, Onandia G, Gessler A (2022). Can forest trees take up and transport nanoplastics?. iForest 15: 128-132. - doi: 10.3832/ifor4021-015
Academic Editor
Werther Guidi Nissim
Paper history
Received: Nov 15, 2021
Accepted: Feb 22, 2022
First online: Apr 09, 2022
Publication Date: Apr 30, 2022
Publication Time: 1.53 months
Copyright Information
© SISEF - The Italian Society of Silviculture and Forest Ecology 2022
Open Access
This article is distributed under the terms of the Creative Commons Attribution-Non Commercial 4.0 International (https://creativecommons.org/licenses/by-nc/4.0/), which permits unrestricted use, distribution, and reproduction in any medium, provided you give appropriate credit to the original author(s) and the source, provide a link to the Creative Commons license, and indicate if changes were made.
Web Metrics
Breakdown by View Type
Article Usage
Total Article Views: 35575
(from publication date up to now)
Breakdown by View Type
HTML Page Views: 28076
Abstract Page Views: 4849
PDF Downloads: 2182
Citation/Reference Downloads: 13
XML Downloads: 455
Web Metrics
Days since publication: 1187
Overall contacts: 35575
Avg. contacts per week: 209.79
Article Citations
Article citations are based on data periodically collected from the Clarivate Web of Science web site
(last update: Mar 2025)
Total number of cites (since 2022): 5
Average cites per year: 1.25
Publication Metrics
by Dimensions ©
Articles citing this article
List of the papers citing this article based on CrossRef Cited-by.
Related Contents
iForest Similar Articles
Research Articles
Bioaccumulation of long-term atmospheric heavy metal pollution within the Carpathian arch: monumental trees and their leaves memoir
vol. 17, pp. 370-377 (online: 27 November 2024)
Review Papers
Monitoring the effects of air pollution on forest condition in Europe: is crown defoliation an adequate indicator?
vol. 3, pp. 86-88 (online: 15 July 2010)
Technical Reports
Air pollution regulations in Turkey and harmonization with the EU legislation
vol. 4, pp. 181-185 (online: 11 August 2011)
Editorials
COST Action FP0903: “Research, monitoring and modelling in the study of climate change and air pollution impacts on forest ecosystems”
vol. 4, pp. 160-161 (online: 11 August 2011)
Research Articles
Long-term monitoring of air pollution effects on selected forest ecosystems in the Bucegi-Piatra Craiului and Retezat Mountains, southern Carpathians (Romania)
vol. 4, pp. 49-60 (online: 05 April 2011)
Research Articles
Dust collection potential and air pollution tolerance indices in some young plant species in arid regions of Iran
vol. 12, pp. 558-564 (online: 17 December 2019)
Research Articles
Growth patterns of Scots pine (Pinus sylvestris L.) under the current regional pollution load in Lithuania
vol. 8, pp. 509-516 (online: 12 November 2014)
Commentaries & Perspectives
Clean air policy under the UNECE Convention on long-range transboundary air pollution: how are monitoring results “translated” to policy action
vol. 2, pp. 49-50 (online: 21 January 2009)
Research Articles
First results of a nation-wide systematic forest condition survey in Turkey
vol. 4, pp. 145-149 (online: 01 June 2011)
Research Articles
A fast screening approach for genetic tolerance to air pollution in Scots pine field tests
vol. 6, pp. 262-267 (online: 01 July 2013)
iForest Database Search
Search By Author
- ME Murazzi
- P Cherubini
- I Brunner
- R Kägi
- M Saurer
- P Ballikaya
- F Hagedorn
- M Al Sid Cheikh
- G Onandia
- A Gessler
Search By Keyword
Google Scholar Search
Citing Articles
Search By Author
- ME Murazzi
- P Cherubini
- I Brunner
- R Kägi
- M Saurer
- P Ballikaya
- F Hagedorn
- M Al Sid Cheikh
- G Onandia
- A Gessler
Search By Keywords
PubMed Search
Search By Author
- ME Murazzi
- P Cherubini
- I Brunner
- R Kägi
- M Saurer
- P Ballikaya
- F Hagedorn
- M Al Sid Cheikh
- G Onandia
- A Gessler
Search By Keyword