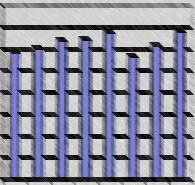
Effects of increasing CO2 on trees and intensively monitored plots: research needs in view of future ecosystem studies
iForest - Biogeosciences and Forestry, Volume 4, Issue 4, Pages 172-175 (2011)
doi: https://doi.org/10.3832/ifor0590-004
Published: Aug 11, 2011 - Copyright © 2011 SISEF
Review Papers
Collection/Special Issue: COST Action FP0903 (2010) - Rome (Italy)
Research, monitoring and modelling in the study of climate change and air pollution impacts on forest ecosystems
Guest Editors: E Paoletti, J-P Tuovinen, N Clarke, G Matteucci, R Matyssek, G Wieser, R Fischer, P Cudlin, N Potocic
Abstract
The intensively monitored plots in Europe have offered a lot of information with regard to the dynamics of forest ecosystems. A large stock of data is already available as an input to ecological models. The carbon sequestration challenge is a little different from others. It requires long-term studies and additional information from what already exists. So far, apart from the determination of organic C in soils, research in the intensively monitored plots has mainly focused on above ground processes, i.e., crown assessment, phenology, deposition, litterfall, tree growth, and foliar chemistry. All these parameters are valuable and will continue to be so. However, according to the latest literature reviews on the subject, the key to understanding the reaction of trees to climate change lies in the dynamics of belowground processes. Information is needed on nitrogen mineralization rates, soil respiration rates and labile carbon forms in soils. If we take into account that countries pay for their carbon emissions and are paid for carbon sequestration, a research like this can be worth doing. Most importantly, the ecological models can be enriched and therefore be more precise in predicting tree response to climate change.
Keywords
Introduction
There is a growing increase in the impact of elevated atmospheric CO2 on forest trees and forest ecosystems. This is not surprising as forests cover some 27% of the total land surface but account for some 70% of terrestrial net primary production ([27]). Moreover, more than 85% of the total plant C on earth and between 60-70% of the total soil C is contained in forests ([10]). The atmospheric concentration of CO2 is currently increasing at the rate of 3.3 billion tons of C per year ([17]). Very good reviews have been published about the consequences of elevated atmospheric CO2 on forests ([5], [34], [6], [19], [23]). Ceulemans et al. ([6]) argue that the question is not so much by what we do not know, but rather how to best integrate our knowledge in order to predict the performance and productivity of future ecosystems to global climatic changes. Newman et al. ([29]) have the opinion that our current ability to detect and predict changes in forest ecosystem productivity is constrained by several limitations. These include a poor understanding of belowground productivity, the short duration of most analyses, and a need for greater examination of species or community-specific variability in productivity studies. From studies to date, we know that the life-long above ground growth response of forest trees cannot be accurately predicted from short-term experiments ([30]). Karnosky ([19]) suggests long-term studies using free-air CO2 enrichment (FACE) technologies or forest stands around natural CO2 vents so as to increase the knowledge base on forest ecosystem responses to elevated atmospheric CO2. There is no doubt that FACE experiments can offer valuable information. However, there are some shortcomings with those experiments. First is the representativity. If the FACE experiments try to cover the whole range of forest ecosystems types, the cost will be tremendously high. The second is that FACE experiments will have a higher rate of CO2 enrichment than the actual rising rate of CO2. Forest stands around natural CO2 vents are rather limited. The establishment of new plots in natural atmosphere has, of course, the disadvantage of the very long-term expectancy of conclusions. Hopefully, there are already experimental plots, which have a past of ecological measurements. These are the intensively monitored plots (800 in total) which were installed in Europe under the auspices of the ICP-Forests ([16]). Those plots were installed when concern about the threat of atmospheric pollution on forest vitality was widespread. In this work I will try to stress the importance of keeping monitoring these plots. More specifically, I will focus on the potential contribution the intensively monitored plots can offer to meet the knowledge gaps with regard to tree responses to elevated atmospheric CO2. These knowledge gaps were set by Karnosky ([19]). The measures suggested concern long-term monitoring so they must be as cost efficient as possible.
Tree growth
It has been found that short-term assimilation of CO2 is significantly stimulated by increased CO2 in nearly all plant species ([34], [25]). However, in the longest study (25-30 years) of continuous exposure of forest trees to elevated atmospheric CO2 with forest stands of holm oak growing in the vicinity of two natural CO2 springs in Italy, it was found that the trees showed a moderate, age dependent increase in stem biomass production, but had significantly lower biomass of 6-year-old branches, decreased branching, and lower leaf area per unit branch biomass, compared to control trees at a nearby site ([13]). In the intensively monitored plots growth has been measured since 1995. At that time tree diameter was measured either with measuring tapes or calipers. Recently, during the Pan European FutMon project (⇒ http://www.futmon.org/) some diameters were measured with girth bands. These bands are relatively cheap and very accurate. This kind of measurement can be extended in all plots to include many more trees. In some years there will be valuable results all over Europe.
Water balance
Long-term studies of forest trees in an enriched atmosphere with CO2 have shown a significant 21% decrease in stomatal conductance ([26]). However, as tree biomass increases the question remains whether trees use more or less water even if stomatal conductance decreases. The installation of soil moisture sensors in many plots help in the calculation of the whole water balance in forest stands. The actual magnitude of transpiration cannot be calculated but a comparison between forest stands can be made. For instance, for the same amounts of rainfall and interception the stand with the less water loss in drainage (assuming there is no surface runoff) will have a higher transpiration rate. The soil water holding capacity of each stand will be a covariate in the statistical comparison.
Soils
An important aspect that has not been tackled so far by the ICP-Forests projects is the form of C in soils. Elevated atmospheric CO2 will bring about a stimulation of soil respiration, which can be much higher than the enhancement of root biomass ([4]). A major consequence of the increase in microbial activity and consequently in CO2 production is a potential negative effect on the accumulation of organic C in soils and thus C potential sequestration of soils ([15]). However, there is a plateau beyond which organic matter cannot be easily attacked by microbes. Mineralization of soil organic matter strongly depends on the size of the labile C pool in addition to the effects of the microbial community and abiotic environmental conditions like temperature and moisture ([7]). A simple method to measure the labile C pool in soils is by water extraction ([18]). Although water extractable organic C makes up only a small portion of total soil organic matter (usually less than 1% - [3]), it might provide a measure of microbially available C. Zhao et al. ([37]) found a strong relationship between water extractable organic C and the rates of C mineralization using arable and forest soils.
In the intensively monitored plots the two soil surveys have a distance of 10 years from each other (1997 and 2007). Most counties, if not all, have stored the samples so they can be reanalyzed. If a third survey takes place in another 10 years, there will be a set of data with the amount of microbially available C covering a time space of 30 years. Comparisons can be made having in mind a fourth or fifth survey for the next generations.
Plant nutrition and nutrient cycling
The growth response of forest plants to the rising concentrations of CO2 depends on their ability to acquire soil nutrients and water ([12]). Nutrient imbalances are reflected in foliar analysis. Every two years foliar analysis is carried out in all plots. A valuable data bank has been formed and should be enriched in the following years.
It has been well documented that the nitrogen level in the foliage of trees growing under elevated atmospheric CO2 generally decreases ([24]). As an example for repeated measures analyses, Fig. 1 shows N concentrations in fir foliage over time in one of the intensively monitored plots in Greece ([28]). In that particular case no significant changes were observed.
Nitrogen concentrations also decrease in litterfall ([31]). By contrast to N concentration, the quantity of litterfall increases 20-30% under elevated CO2 ([9]). It is not known how nutrient mineralization will change due to the higher quantity of CO2 in soil. In the intensively monitored plots litterfall is monitored. Litterfall quantities are measured and elemental analyses are carried out. A simple test is a time series comparison between the successive N concentrations in foliage and foliar litterfall.
If the N content in litterfall decreases so must decrease the litter decomposition rates and the N mineralization rates. Both of them can be measured with the classic method of the litterbags technique. This is a relatively easy technique that can be employed in the future.
Belowground carbon allocation
Allocation of C to belowground plant structures often equals or exceeds aboveground litterfall C and aboveground respiration in forest ecosystems, making it the single most important fate for gross primary productivity ([20]). Despite its importance, total belowground allocation (TBCA) remains poorly quantified because it is difficult to quantify root and mycorrhizal processes by any method ([11], [14]). If growth is enhanced, there will be more C input into the soil. Therefore, a major indirect response to an increase in atmospheric CO2 consists in the greater below-ground C allocation through root exudation and turnover, which is likely to lead to changes in the size and the activity of soil microflora ([22]).
In the absence of direct measurements of TBCA, Raich & Nadelhoffer ([33]) and Davidson et al. ([8]) proposed the following equation (eqn. 1):
where soil respiration is the sum of root respiration, root litter C decomposition and aboveground litter C decomposition.
A critical assumption of this approach to estimate TBCA is that that the ecosystem is at a steady state, which means that the annual inputs of C below ground are equal to annual rates of decomposition.
If the system is not at a steady state the previous equation becomes (eqn. 2):
where ΔC
soil, ΔC
litter, ΔC
root are the changes in C stocks of mineral soil, forest floor and root biomass, respectively, and C
xport is C loss through leaching. From the above equation the parameters R
soil, C
litterfall and C
export in soil solution can be measured continuously in the intensively monitored plots. ΔC
soil and ΔC
litter can be measured in the following soil survey and compared with the survey in 2007. ΔC root can be calculated from an equation between ABD
(aboveground biomass density) in Mg ha-1 and RBD
(root biomass density) also in Mg ha-1.
Cairns et al. ([2]) used the following equation (eqn. 3):
The above equation was based on 160 studies covering tropical temperate and boreal forests. The use of this equation is encouraged by other workers ([1]).
The total above ground biomass includes foliage apart from woody biomass. There are equations connecting foliage and tree diameters and heights for the main forest species ([21]), so that all components of the equation can be found.
In order to apply the above equations, the soil respiration rates have to be measured. It is probably the only expensive measurement, but it will help in drawing valuable conclusions, as soil respiration depends on soil moisture and temperature repeated measures have to be taken in time and space. Vincent et al. ([35]) used soil respiration chambers on which a portable infrared gas analyser was connected. They measured respiration in daytime during the growth period in a temperate deciduous forest. Raich ([32]) measured soil respiration with the soda-lime technique in three Hawaiian rain forests in a whole year period. The soil respiration fluxes should be expressed as the littefall fluxes, i.e., in kg ha-1 yr-1. So a whole year of measurements is necessary. The number of samplers (chambers) depends on the variability of respiration rates and the required probability level. A trial can be carried out with a limited number of samplers after which the required sample size for a given confidence interval can be calculated according to statistical formulas ([36]).
In my opinion, the first simpler equation may be used in most cases and the second one may be applied when there is a third soil survey in the intensively monitored plots.
Phenology
Phenology is the study of the periodicity of leafing, flowering and fruiting in plants in relation to climate and other environmental factors. There is the hypothesis that elevated CO2 can affect development of leaf area in the spring so that trees could potentially benefit from an earlier onset of C assimilation at the start of the growing season. This could be an important factor in the expansion of tree populations into areas currently too cold for their growth ([34]). Phenological observations are carried out regularly in the intensively monitored plots and if leaf area increases that will be written down. What I now suggest is the extension of phenological observations to mycorrhizal fungi. This is crucial because the plants’s ability to acquire soil resources in an elevated CO2 environment is mediated by symbiotic associations with mycorrhizal fungi. A new distribution of mycorrhizal fungi may not be beneficial to plants as the plant-fungi symbiosis was evolved over time due to natural selection.
Conclusions
The intensively monitored plot can be a valuable tool in assessing the impact of elevated CO2 on forests. The activities already existing should be complemented by the following actions:
- Tree growth is suggested to be monitored with girth band in all plots;
- A third soil survey must be carried out. In this survey, together with stored samples of the previous ones, the labile C should be determined by water extraction.
- Soil respiration must be measured so as to draw conclusions on the C allocated belowground.
- Organic matter mineralization rates should be determined in all plots.
- Phenology observations must include the distribution of mycorrhizal fungi.
References
CrossRef | Gscholar
Gscholar
Gscholar
Gscholar
Gscholar
Authors’ Info
Authors’ Affiliation
Forest Research Institute of Athens, Terma Alkmanos, GR-115 28 Athens (Greece)
Corresponding author
Paper Info
Citation
Michopoulos P (2011). Effects of increasing CO2 on trees and intensively monitored plots: research needs in view of future ecosystem studies. iForest 4: 172-175. - doi: 10.3832/ifor0590-004
Paper history
Received: Nov 25, 2010
Accepted: Mar 09, 2011
First online: Aug 11, 2011
Publication Date: Aug 11, 2011
Publication Time: 5.17 months
Copyright Information
© SISEF - The Italian Society of Silviculture and Forest Ecology 2011
Open Access
This article is distributed under the terms of the Creative Commons Attribution-Non Commercial 4.0 International (https://creativecommons.org/licenses/by-nc/4.0/), which permits unrestricted use, distribution, and reproduction in any medium, provided you give appropriate credit to the original author(s) and the source, provide a link to the Creative Commons license, and indicate if changes were made.
Web Metrics
Breakdown by View Type
Article Usage
Total Article Views: 52180
(from publication date up to now)
Breakdown by View Type
HTML Page Views: 44755
Abstract Page Views: 2539
PDF Downloads: 3498
Citation/Reference Downloads: 11
XML Downloads: 1377
Web Metrics
Days since publication: 5088
Overall contacts: 52180
Avg. contacts per week: 71.79
Article Citations
Article citations are based on data periodically collected from the Clarivate Web of Science web site
(last update: Mar 2025)
Total number of cites (since 2011): 2
Average cites per year: 0.13
Publication Metrics
by Dimensions ©
Articles citing this article
List of the papers citing this article based on CrossRef Cited-by.
Related Contents
iForest Similar Articles
Research Articles
Predicting the effect of climate change on tree species abundance and distribution at a regional scale
vol. 1, pp. 132-139 (online: 27 August 2008)
Research Articles
Seeing, believing, acting: climate change attitudes and adaptation of Hungarian forest managers
vol. 15, pp. 509-518 (online: 14 December 2022)
Research Articles
Potential impacts of regional climate change on site productivity of Larix olgensis plantations in northeast China
vol. 8, pp. 642-651 (online: 02 March 2015)
Editorials
Future monitoring and research needs for forest ecosystems in a changing environment: an introduction
vol. 2, pp. 54-55 (online: 21 January 2009)
Research Articles
Impact of climate change on radial growth of Siberian spruce and Scots pine in North-western Russia
vol. 1, pp. 13-21 (online: 28 February 2008)
Review Papers
Impacts of climate change on the establishment, distribution, growth and mortality of Swiss stone pine (Pinus cembra L.)
vol. 3, pp. 82-85 (online: 15 July 2010)
Research Articles
Contrasting multi-taxa diversity patterns between abandoned and non-intensively managed forests in the southern Dolomites
vol. 10, pp. 845-850 (online: 26 October 2017)
Research Articles
Acid atmospheric deposition in a forested mountain catchment
vol. 10, pp. 680-686 (online: 17 July 2017)
Research Articles
An assessment of climate change impacts on the tropical forests of Central America using the Holdridge Life Zone (HLZ) land classification system
vol. 6, pp. 183-189 (online: 08 May 2013)
Research Articles
Approaches to classifying and restoring degraded tropical forests for the anticipated REDD+ climate change mitigation mechanism
vol. 4, pp. 1-6 (online: 27 January 2011)
iForest Database Search
Search By Author
Search By Keyword
Google Scholar Search
Citing Articles
Search By Author
Search By Keywords