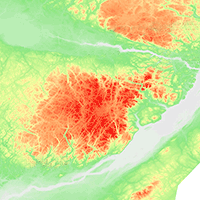
Measuring natural sap production in sugar maple at daily temporal resolution
iForest - Biogeosciences and Forestry, Volume 17, Issue 5, Pages 323-330 (2024)
doi: https://doi.org/10.3832/ifor4591-017
Published: Oct 19, 2024 - Copyright © 2024 SISEF
Research Articles
Abstract
Sap yield for maple syrup production is well studied at annual scale. However, the phenological timings of sap production have been less explored. Our study tested the use of rain gauges for monitoring timings and dynamics of sap production at daily temporal resolution. The batteries of the rain gauges were tested under controlled conditions at temperatures ranging from -20 to 5 °C and logging intervals of 15 and 30 minutes. In 2022, eight rain gauges were installed in the field on maples located in four sites along a latitudinal gradient in Quebec, Canada. The batteries performed well at all temperatures in both field and controlled conditions, showing a higher state of charge at logging intervals of 30 minutes with temperatures warmer than -15 °C. The dynamics of sap exudation were correlated among and within sites, demonstrating that trees respond to common environmental factors at regional scale. The natural sap production was discontinuous, alternating productive and non-productive days. Overall, 74% of the sap was exudated during 20% of the sugar season, which matched the proportions described by the Pareto law. Such a heterogeneous and unbalanced distribution in sap exudation exposes small producers to challenges for the use of equipment and makes maple syrup production sensitive to climate hazards in a context of climate change.
Keywords
Acer saccharum Marsh., State of Charge, Rain Gauge, Sap Exudation, Sap Yield, Tipping Bucket
Introduction
Maple syrup is one of Canada’s leading products for exportation and the Quebec region alone has 90% of the total sap yield, producing around 72.2 million litres in 2022, an increase of 59.1% from 2021 ([37]). However, sap yield is exposed to huge variations. Sap yield in 2021 dropped by 24% compared to 2020. These interannual variations mainly come from the particular and specific set of meteorological conditions needed to collected sap from the sugar maple stems. Sap exudation depends on specific daily thermal ranges, from -10 °C to 13 °C for the onset and -6 °C to 7 °C for the end of the sap season, and drops sharply with minimum temperatures < -8 °C ([33], [22]). Basically, the period time of these occurrences for these daily ranges and the number of their occurrences define respectively, the sugar season, and its yield. Although it is known that such a thermal variation is necessary for sap exudation, there is still a lack of information on the mechanisms occurring within the tree. While the total sap yield is known at annual scale ([31], [33]), the pattern of sap production at higher temporal resolution has historically been less explored, due to the lack of suitable equipment. Information on daily and hourly production patterns could provide new insight into the eco-physiological processes acting at tree scale and have a great logistic interest for producers.
The sap yield reported in official records are obtained through annual surveys by consultants or from online platforms, using input coming directly from local producers ([8], [5]). Responses to the survey can include information about location, number of tapped trees and the producers, but maple syrup production is provided exclusively in terms of total sap yield ([8], [5]). The volume of sap is collected in reservoirs and the seasonal estimate of the total volume is calculated. Total yield is determined by the ratio of annual production to the number of taps ([8]).
In a pioneer study performed in 1956, Marvin & Erickson ([27]) measured the sap volume using a tipping bucket with a balance that held 15 ml at a time when it was completely full. When the bucket tilted to empty, an impulse was sent to a data logger, which recorded it on a graph at the same speed as the overturning and thus with low accuracy, due to the limitations of the technology at that time. Despite their work being focused on the effect of environmental factors (i.e., temperature and its fluctuation) on sap production, contemporary equipment such as the modern rain gauge could represent a reliable tool to monitor sap production at tree scale, and daily temporal resolution.
A daily or hourly temporal resolution can be a solution to reach precise estimations of sap production. To obtain sap volume measurements at high temporal resolution, automatic tools precisely recording the hourly volumetric flow should be employed. As an example of automatic tool, the rain gauge is traditionally used to record rainfall intensity and duration of rain events ([38], [45]). Currently, it is widely used due to their adaptability to different data loggers, easy manipulation and because they provide precise measurements, allowing accurate records of local precipitation events to be obtained ([39]). The data collected by rain gauges can be registered at specific intervals, such as minutes or hours. The measurement is done with rain gauges recording a minimum volume of 3.73 ml of water, which can be used to precisely assess rates, times and duration of a rainfall event ([28]). Tipping bucket rain gauges could potentially represent an effective solution for monitoring sap production at high resolution temporal scale.
There is a need to quantify sap exudation during the sap season by means of specific or adapted measuring tools, e.g., using the tipping bucket rain gauge. To this end, we investigate the ability of rain gauge to operate under the harsh conditions typical of the spring of maple, where temperatures can drop below -20 °C, a possible primary constraint for the use of this equipment ([3]). The data logger connected to the rain gauge is indeed supported by lithium ion (Li-ion) batteries, whose performance and lifespan can be reduced by temperatures above or below a certain recommended range ([19]). Recently, studies have focused on the responses of a Li-ion battery to temperature, as this plays a crucial role in getting the batteries to run at maximum efficiency ([16], [2], [3]). Most Li-ion batteries perform well between 15 °C and 35 °C, near room temperature ([20]). Batteries lose 1.5% to 2% of their capacity and 10% of their power capabilities when the temperature varies by 5 °C ([9]). Tipping bucket rain gauge could also be affected by the biochemical composition of maple sap. Recording the volume of an aqueous solution, such as maple sap, that contains low concentrations of reducing sugars, such as glucose, fructose, and xylose ([23]) could be a challenge for the measurements by potentially sticking the pivot mechanism of the tipping buckets.
The aim of this study is to test the use of the rain gauge as a tool for monitoring the timings and dynamics of sap production in sugar maple. We firstly tested the performance of the batteries employed in the data logger in controlled conditions under a range of temperatures occurring during the spring. Subsequently, we tested the rain gauge effectiveness in measuring sap volume in natural conditions during the sugar season and by assessing the correlation in sap production between and within sites along a latitudinal gradient in Quebec, Canada. We expected that a shorter logging interval or colder conditions would drain more battery than longer intervals or measurements realized under warmer thermal conditions.
Materials and methods
Testing the batteries of rain gauges under controlled conditions
Tipping bucket rain gauges (HOBO Data Logging Rain Gauge RG3-M®, Bourne, MA, USA) were exposed to four constant temperatures (-20, -15, -10 and +5 °C) in controlled conditions (i.e., room fridge and freezer) recorded at 15 and 30 minutes. This range in temperature covered the thermal conditions experienced during the sugar season, from mid-February to the end of April, in Quebec, Canada ([22]). Two rain gauges equipped with a new lithium battery (CR-2032 Lithium 3V, Sony, Japan) were installed at each thermal condition and set up to record data at a logging interval of 15 or 30 minutes. The state of charge was recorded every two days at the beginning of the experiment and weekly when it reached a stable level. State of charge was recorded using the Hobo software (Hoboware software, Bourne, MA, USA) by connecting a data logger to a laptop through a coupler and collecting the measurements after 10 minutes. The rain gauges have a time accuracy of ± 1 minute per month at 25 °C, and collect data under a thermal interval ranging between -20 °C and 70 °C with an accuracy of ± 0.54 °C from 0 °C to 50 °C. The logger measured the state of charge with a resolution ranging between 3% and 7% ([28]).
Monitoring sap production in the field
Four study sites, Rivière-à-Pierre (abbreviated as RAP), L’Anse-Saint-Jean (ASJ), Laterrière (LAT), and Parc National de Monts-Valin (PMV) were selected in Quebec, Canada, at the northern part of sugar maple distribution in northeastern North-America (Fig. 1). RAP (46° 91′ N, 72° 03′ W) is located in the hardwood forest subzone belonging to the sugar maple-basswood domain. ASJ (48° 19′ N, 70° 22′ W), LAT (48° 28′ N, 71° 14′ W) and PMV (48° 61′ N, 70° 79′ W) are located in the mixed forest subzone belonging to the balsam fir-yellow birch domain. PMV hosts the northernmost stand of sugar maple in North America. The sites cover a range in annual temperature of between 1.3 °C and 3.9 °C (Tab. 1).
Tab. 1 - Location and climate of the four study sites in Quebec. (DBH): stem diameter at breast height.
ID | Site | Elevation (m a.s.l.) |
Annual Temp (°C) |
Annual Rainfall (mm) |
DBH (cm) | |
---|---|---|---|---|---|---|
Tree 1 | Tree 2 | |||||
RAP | Rivière-à-Pierre | 201 | 3.9 | 1325 | 34 | 34 |
ASJ | L’Anse-Saint-Jean | 138 | 2.0 | 1221 | 28 | 33 |
LAT | Laterrière | 200 | 3.5 | 1160 | 37 | 29 |
PMV | Monts-Valin | 926 | 1.3 | 1446 | 22 | 16 |
Eight rain gauges were used to assess timings and dynamics of natural sap production in tapped trees during spring 2022. We selected adult sugar maples, two per site equipped with rain gauges. We recorded tree characteristics as well as the daily air temperatures occurring during the sap season (see Tab. S1 and Fig. S1 in Supplementary material). A notch was made on the stem at 2 m from the ground using a drill following the last tapping procedures. A plastic spout was inserted into the notch and connected by tubing to the rain gauge. The rain gauge was covered and sealed to avoid rain, snow and debris from falling in or otherwise obstructing the measurement system. The data were recorded continuously by the data logger throughout the entire sugar season. The rain gauges were installed before the sugar season (from late February to late March) and removed at the end of it (from early May to early June) according to the weather conditions of the sites ([22]).
Data analysis and statistics
The state of charge quantified in percentage from 0 % (uncharged) to 100% (fully charged), was fitted using a nonlinear least squares regression to describe the variation in time during the experiment. The exponential decay model was defined as follows (eqn. 1):
where y represents the state of charge and t the day of experiment. The three coefficients represent the lower asymptote (a), the vertical amplitude of the curve (b) and rate of battery decay (c). The curve fitting was performed at each temperature and logging interval (i.e., every 15 and 30 minutes) in the controlled conditions. Starting values were specified for each parameter and optimized using the Gauss-Newton algorithm by minimizing the sum of squares errors ([4]). Standardized residuals were checked for fitting the curve. The analysis was performed using the function “nls” implemented in the package “stats” in R version 4.2.1 (R Development Core Team, Vienna, Austria).
Volumes recorded by rain gauges were corrected using a conversion rate of 1.32 g ml-1 to consider the higher density of the sap ([8]). Indeed, each tipping bucket records 3.73 ml and it is equivalent to the sap volume of 2.83 ml, representing a reduction of 24% in the measurement compared to the density of water (1 g ml-1). Sap production could be monitored precisely through the process of calibration and conversion to the appropriate density units.
Cumulative distribution functions were used for assessing the temporal variations in sap production. The cumulative percentage of the data organized in descending order of daily sap production measurements was calculated and plotted against the cumulative percentage of elapsed days. It allows a visual comparison of the amount of sap production along the season at a daily resolution and the frequency of productive days. The daily sums of sap production, expressed as day of the year (DOY), were calculated. Correlations using the non-parametric Spearman coefficient (ρ) were assessed within site (i.e., between trees), and between sites (i.e., between the averages of trees from each site). Correlations among trees or sites were calculated for the period of sap production. All statistics were performed using R version 4.2.1 (R Development Core Team, Vienna, Austria).
Results
State of charge under controlled conditions
The experiment of state of charge in batteries lasted 110 days. State of charge showed a negative exponential shape, with a drop occurring within the first days from the beginning of the experiment (days 10 to 20), followed by stable values (Fig. 2). Rain gauges showed starting state of charge at 100%, except those exposed to -10 °C, which started at 63%. Despite starting with lower state of charge, the batteries exposed to -10 °C reached the plateau after a similar number of days than the other batteries exposed to other temperatures. Logging intervals of 30 minutes showed the highest state of charge except at -20 °C comparing to the logging intervals of 15 minutes. The opposite pattern occurred for the temperature of -20 °C, which showed the lowest state of charge when recorded each 30 minutes. In general, the batteries exposed to warmer temperatures had a higher state of charge. The variation in the state of charge was similar between the two logging intervals. Accordingly, the two batteries exposed to the same temperature reached 50% of their charge during the same period but at different day of experiment.
Fig. 2 - Variation in the state of charge in rain gauges exposed to different temperatures and set at two logging intervals. Data were fitted by nonlinear exponential decay models, whose standardized residuals (SR) are shown in the smaller panels.
Curve fitting of the state of charge
The quality of the fitting was confirmed by analysis of the residuals, which were uniformly distributed for all rain gauges. Most of the standardized residuals of these eight models converged from -2 to 2, confirming the model reliability (Fig. 2). No apparent trend was observed for the residuals, confirming that the model well represented the data. The residuals exhibited a certain heteroscedasticity, with large residuals being concentrated during the first 20 days of the experiment. Overall, the results suggest that the model could be considered suitable for the observed changes in time of the state of charge.
Comparing the coefficients of the models
The nonlinear models produced coefficients varying within similar ranges (Tab. 2). The coefficient a represents the stability (i.e., constant battery status) of the state of charge. The 30-minute logging interval showed higher values of state of charge than the 15-minute logging interval, except when exposed to a temperature of -20 °C where the order was reversed. The lowest value was observed at -15 °C and logging interval of 15 minutes. The highest value was calculated at +5 °C and logging interval of 30 minutes. The coefficient b represents the amplitude of the state of charge and showed the highest values with the logging interval of 30 minutes. The lowest value of the coefficient b was observed at -10 °C and logging interval of 30 minutes. The highest value was detected at -15 °C and logging interval of 15 minutes. The coefficient c indicated the speed to the battery drop or the rate of battery decline, and that the battery ran out faster at -10 °C and logging interval of 30 minutes. The lower value was observed at -15 and -20 °C (logging interval of 30 minutes - Tab. 2).
Tab. 2 - Estimated coefficients and statistics of the nonlinear models describing the variation in the state of charge of rain gauges submitted to different temperatures and set up at different logging intervals. Values are reported as a mean ± standard error.
Temperature (°C) | Logging interval (minutes) |
a | b | c |
---|---|---|---|---|
5 | 15 | 51.58 ± 0.71 | 50.10 ± 3.49 | -0.28 ± 0.04 |
5 | 30 | 55.44 ± 0.42 | 46.18 ± 1.98 | -0.33 ± 0.03 |
-10 | 15 | 13.67 ± 0.81 | 50.27 ± 4.38 | -0.47 ± 0.09 |
-10 | 30 | 34.15 ± 1.19 | 28.85 ± 6.79 | -1.33 ± 1.68 |
-15 | 15 | 16.28 ± 0.88 | 86.59 ± 4.21 | -0.25 ± 0.02 |
-15 | 30 | 35.64 ± 1.54 | 67.26 ± 8.28 | -0.43 ± 0.11 |
-20 | 15 | 25.32 ± 0.89 | 72.53 ± 4.17 | -0.24 ± 0.03 |
-20 | 30 | 18.95 ± 0.75 | 81.85 ± 3.85 | -0.34 ± 0.03 |
Rain gauges in the field
We recorded the daily volume of sap production at the four sites between DOY 80 and 130. Sap production was discontinuous, as demonstrated during the period from DOY 104 to 112 at all sites (Fig. 3). We observed days with high production and days with low or no production. High productions occurred either on single days or during periods, as detected during DOY 88 and 96 at RAP. Low or no production was observed between DOY 108 and 110 at all sites.
Fig. 3 - Daily dynamics of natural sap production in sugar maple and the correlation coefficient of Spearman in each site. Note the different ranges of vertical axes.
The diameter at breast height of the trees varied from 16 to 37 cm, with a height of between 7.5 to 18 m. The maximum average temperatures varied between 2.8 and 3.6 °C, with the warmer site being RIV (Tab. S1 and Fig. S1 in Supplementary material). The minimum average temperatures ranged from 2.2 to 2.9 °C, with the lowest minimum being recorded in ASJ (Tab. S1 and Fig. S1 in Supplementary Material). The highest correlation between trees was observed at ASJ (ρ = 0.88, p<0.001 - Fig. 3). One of the trees at RAP had a short season of sap production, from DOY 80 to DOY 115, which caused a low correlation between trees (ρ = 0.38, p>0.05). The earliest onsets of sap production were observed at PMV and RAP (DOY 80). The latest onset of sap production was detected at ASJ (DOY 95). The earliest ending of sap production was observed at RAP (DOY 115) and the latest ending at LAT (DOY 130). The total yield varied between trees and sites. The highest volume in one day occurred at PMV, with a total yield of 4.5 litres for tree 1.
In general, sap production showed a spatial autocorrelation, where sites close to each other showed higher correlations. For example, ASJ and LAT showed a higher correlation (ρ =0.72, p<0.001) compared to RAP (Tab. 3). The correlation between RAP and ASJ, and between RAP and LAT were not significant (ρ ranging between 0.25 and 0.27, p>0.05). PMV was the only site showing significant correlations with all studied sites (ρ ranging between 0.32 and 0.44, p<0.05).
Tab. 3 - Spearman’s correlation coefficients (ρ) and relative probabilities of correlations in daily sap production during spring 2022 among the four study sites. (NS): not significant.
Site | RAP | ASJ | LAT | PMV |
---|---|---|---|---|
RAP | - | 0.27 | 0.25 | 0.43 |
ASJ | NS | - | 0.72 | 0.32 |
LAT | NS | p<0.001 | - | 0.44 |
PMV | p<0.01 | p<0.05 | p<0.01 | - |
Cumulative percentage of daily sap production
The cumulative percentage of daily sap production showed an exponential decline. Such a pattern indicated that a substantial amount of sap production occurred within a few, very productive days. These productive days are located at the beginning of the curves shown in Fig. 4until the identity line (line 1:1). Most days of the sap season (in the middle and right part of the plots) had low or no sap production. The patterns are very similar between trees and sites despite the differences observed in the absolute amount of sap production, which indicates a common trend within and across regions. For example, the trees at RAP showed a different pattern of volume and period of sap production compared to other sites (Fig. 3). Yet, the cumulative percentages were very similar to the other sites and trees (Fig. 4).
The duration of sap production varied from 35 to 50 days. An average of 74% (from 62.2% to 84.6%) of the total sap production was exuded on eight very productive days (7 to 10 days), which corresponded to 20.1% (19.4% to 20.9%) of the sugar season. Most of the days (14 to 35 days) had low sap production, below 1 litre. No sap production was observed during 6 to 14 days, which corresponded to 23.7 % (16.6 to 40%) of the sugar season.
Discussion
Our study utilized rain gauges to monitor sap production in maple at a daily temporal resolution. To confirm the use of rain gauges as an adequate tool to measure sap production, we conducted tests under controlled conditions and in the field. The rain gauges demonstrated the ability to record data across a wide range of temperatures in the field and continued to log data for over three months under controlled conditions, what is largely exceeding the duration of the sugar season. Our results demonstrated that the rain gauges can remain active at temperatures of -20 °C despite sap exudation will not occur at that low temperature. Field measurements allowed the phenological timings of production to be compared among and within sites. Our findings showed the particular dynamics of sap exudation, which is concentrated in a few, and highly productive, days of the sugar season.
Rain gauge as a tool for measuring sap production
The performance of batteries is known to decline as the temperature falls below 0 °C. According to the coefficient a of our model, the batteries showed a higher stability at temperatures > 0 °C, and a lower stability at temperatures < -10 °C (Tab. 2). According to previous studies, batteries exposed to -10 °C exhibit a reduction in capacity by up to 95% compared to those exposed to ambient temperature (i.e., 20 °C), which can explain such low battery status in the coefficient a values ([47]). The coefficient c showed a faster battery decline. This is due to several factors, including slower chemical reactions, reduced rates of battery transfer kinetics, and decreased electrolyte conductivity, which limit the diffusivity of lithium ions in a negative electrode potential, making it easier to oxidize ([35], [46], [16]). At -20 °C, the extraction of energy is significantly limited and energy and power of the cells decrease, resulting in lower battery-transfer resistance ([47]). These opposing forces can negatively impact the performance of the battery during its operation ([17], [47]).
The Lithium batteries were suitable for dataloggers running at low temperatures. The supply voltage of Li-Battery is 3V, and the input resistance of the datalogger is 100 kΩ, as mentioned in the datasheet of the datalogger ([28]). Using Ohm’s Law, the value of current is 3V/100 kΩ = 0.03 mA, ten times less than the rated current of the battery (0.3 mA for continuous drain according to the datasheet of Sony Li-Battery CR2032). Hence, a fairly low current is drawn from the battery by the datalogger. Even under a constant temperature of -20 °C, the rain gauge was able to record data for 110 days during the experiment. Except for very low temperatures, the time interval of 30 minutes was the best resolution to record data while maintaining a higher state of charge. However, a resolution of 15 minutes was more effective at -20 °C to ensure a long-lasting performance of the battery. Lithium batteries should undergo pulse discharge when exposed to extreme temperatures, which can promote a better performance, even if lower temperatures result in minimal change compared to -10 °C.
Our experiment showed that the batteries performed better and had a higher battery level when they were used less frequently, i.e., 30 minutes (Fig. 2). Using the battery less frequently allowed voltage to rise and to maintain a higher battery level. However, at -20 °C, the results were reversed, with the battery performance being higher when the battery was used more often. When the temperature is below 0 °C, battery discharging warms up the cells due to an interaction between electrochemical and thermal processes ([15]). The self-heating process contributes to maintain the state of charge stable over time ([15]). At -20 °C, a shorter logging interval, i.e., 15 minutes, warms up the battery more frequently, likely contributing to maintaining a higher state of charge than a longer logging interval, i.e., 30 minutes. Nevertheless, a shorter logging interval, and consequently a more frequent heating of the cell system, can cause a quicker deterioration of cells, reducing the battery lifespan ([26], [15]).
The high input/output impedance (a low current flow in a circuit and potentially high voltage) of 100 kΩ of the data logger, combined with the short duration of current flow in a circuit of the battery, reduces the current requirement to maintain the battery functioning properly and significantly prolongs the battery life. In fact, our setup was able to use a CR-2032 3V lithium battery for the entire period of the experiment, exceeding the sap production season, which on average lasts 8 to 10 weeks ([22]). As a result, the data logger can easily operate with the CR-2032 3V lithium battery for the entire sap production season without the need for battery replacements.
Overall, our experiment in controlled conditions demonstrates the rain gauge’s ability to function under extreme temperatures for the whole sap season, making it a reliable tool for recording sap production in maple trees at high temporal resolution.
Dynamics of sap exudation and sap yield
The rain gauges were used in the field to assess sap exudation at high temporal resolution and validate the performance under natural conditions. The tool enabled accurate measurements and comparisons of sap production throughout the entire sugar season. The phenological timings during the sap season (i.e., the sap flow in late winter and early spring when temperatures fluctuate) were correlated between the study sites, which could be attributed to the similar climatic conditions that the maple population experienced across sites. Indeed, the period of syrup production as well as the annual yield are known to be strongly climate-driven ([33]). In our study, we observed that the southernmost and warmest site (RAP) concentrated most sap production at the end of March (DOY 88) and beginning of April (DOY 98). The other sites, ASJ, LAT and PMV, showed the highest sap volume occurring in mid-April (DOY 108-112). This difference in timing between sites could be explained by the earlier warming occurring at the southern site (RAP), which experienced the ideal conditions for growth reactivation earlier than the northern sites, leading to an earlier onset of the sugar season. The later onset of sap production was observed at ASJ, possibly because of its cooler temperature compared to the other sites. Thermal thresholds, above and below 0 °C play a critical role in triggering sap exudation, and the specific conditions required to initiate the sugar season are well documented ([36], [33]). In North America, this season typically occurs within a specific timeframe in late winter and early spring ([14]). The northern sites, located in the same climatic zone and experiencing similar weather, showed similar timings of sap exudation, with trees within the same site exhibiting comparable onset, ending and duration of the sugar season ([22]). This result suggests that measurements collected in a few individuals could be sufficient to obtain a precise picture of the sugar season at site and regional scale. However, further investigations with a larger sample size are recommended to assess more accurately and extensively the variability in phenological timings among trees. The results suggest the need to evaluate carefully the minimum sample size for estimating the sap yield for the future experiments. Moreover, given the disparities in density between rainwater and sap , and the potential variations in sap density throughout the season ([29]), it is advisable to conduct additional research to better relate sap volume to its density. Such a study would not only fulfil the practical necessity of accurately calibrating the rain gauge for fine-scale sap exudate measurement but could also yield insights into the underlying physiological processes contributing to sap density fluctuations during the sugar season.
Our results demonstrate clearly that sap production is discontinuous, showing either productive or non-productive days during the season. It is known that sap exudation occurs when trees experience freeze-thaw cycles, which are produced by the freezing nighttime temperatures followed by daytime warmings that induce alternating positive and negative pressures in the trunk and branches ([41], [21], [42], [6], [11]). The absence of sap production may be related to daytime temperatures < 0 °C or by low daily amplitudes in temperature ([44]). While the freeze-thaw cycle plays a major role in the sap exudation process, other factors can account for the variation in productivity (i.e., more or less productive days), including environmental parameters such as maximum temperature during the day, water availability and solar radiation ([43], [21], [30], [34]).
According to our results, trees from the same site show similar phenological timings and recurrence of productive and non-productive days, but very different daily and seasonal volumes of sap production. Some factors related to tree characteristics are known to be involved in sap yield. Trees with greater diameters ([13]) and crown size ([40]), or higher vitality can have higher sap yields ([31]). Reproduction effort of sugar maple has also been correlated to syrup production ([32]). Moreover, anatomical differences (e.g., the proportions of rays’ cells) between trees may also explain part of the differences in sap yield ([12]). Still, several sources of variability such as the tapping procedure or tapping location along the stem could affect the final production ([31]). Additional research is needed to better understand the dynamics of sap production at higher temporal resolutions (hourly or sub-hourly) and compare sap exudation with the production using a vacuum system.
Daily distribution of sap exudation
On average, 74% of the total sap yield was produced during 20% of the sugar season. Such a pattern matches the proportion described in economic studies, and referred to as the Pareto law, or 80/20 rule ([18]). This principle, stating that 80% of consequences come from 20% of causes, is relevant to understand the processes involved in productive systems and their optimisation ([7]). It also applies to sap production, with noteworthy implications for producers and their strategic choices for the sugar farm. A possible explanation for this pattern may be related to either the weather conditions during or preceding the harvesting season ([27], [8]). A prompt and reliable use of equipment and human resources is vital during these few, very productive days to ensure an optimal sap collection ([29]). Equipment involves the correct functioning of the tubing system, which should avoid leakages, and losses of vacuum, which can negatively influence the sap flow and consequently the overall sap yield during the most productive days ([24]). A strategy is needed to plan for the peaks in production under the conditions of the 80/20 rule. Producers should ensure an adequate storage capacity for the large amount of sap that can be collected during the most productive days, and the necessary human resources to handle the increased volume of sap ([29]). Statistics of sap production are commonly available at annual scale in Quebec, and there is a need of collecting and analysing sap production at higher resolution for exploring the eco-physiological processes involved in sap exudation driven by the weather ([1], [31]).
The ongoing climate change raise concerns about the future of the maple syrup industry ([10], [33]). Warming winter and spring temperatures might anticipate the onset of sap exudation, forcing producers to modify the traditional tapping schedules ([36]). Changes in the frequency of freeze and thaw events ([33]) could in turn affect the occurrence of the most productive days, with potential consequences on the daily sap yield. According to our results, given that up to 84.6% of the production is concentrated in 20% of the sugar season, changes in weather occurring during the most productive days and even more so the disappearance of the weather conditions creating the most productive days will result in a significant alteration of the seasonal sap yield. There is a need to increase knowledge on the timings, frequency, and intensity of weather events under climate change scenarios to better understand the consequences for sap production and the future of the maple syrup industry ([22], [25]). Data collected at daily temporal resolution using rain gauges allow our understanding of the dynamics of sap exudation to be improved, thus contributing to optimizing the production practices based on the Pareto principle and ensure long-term sustainability of the maple syrup industry.
Conclusions
Our study has developed and tested the use of rain gauges for recording sap production in maple trees at a high temporal resolution. Our findings demonstrate that tipping bucket rain gauges can effectively perform under the harsh spring temperatures typical of the sugar season, including the extreme conditions of -20 °C. It has shown the ability to log data for extended periods, exceeding the typical duration of the sugar season. The results have demonstrated daily synchronisms in sap exudation among and within sites, showing the typical pattern described by the Pareto principle. The sugar season was discontinuous in all sites and exhibited both productive and non-productive days with most of the production being concentrated in 7-10 days. The synchronism in phenological timings among trees presents better than the different daily and seasonal volumes in sap yield.
Our results demonstrated that rain gauges are a reliable tool for monitoring sap exudation with high temporal resolution, offering a new method to deepen our quantitative understanding of the factors driving the sugar season and sap production. By associating the dynamics of sap production with weather data, new insights into the eco-physiological processes involved in sap exudation could be provided. These insights can directly impact the maple syrup industry by enabling producers to manage resources more efficiently and plan the collection of sap in order to better profit from the peak of production.
Acknowledgements
SK carried out the conceptualization, methodology, writing original draft preparation, writing-reviewing and editing. GB carried out the methodology, writing original draft preparation, writing-reviewing and editing. GS carried out the methodology, formal analysis, writing original draft preparation, writing-reviewing and editing. RS carried out the writing original draft preparation, writing-reviewing and editing. SD carried out writing-reviewing and editing. SR carried out the conceptualization, writing-reviewing, editing and supervision.
The authors thank A. Gauthier, S. Néron, V. Néron, A. Roussel, R. Gagné, and the Parc des Monts Valin for permitting the study on their property, and A. Garside for checking the English text.
This research was funded by Conseil de recherches en sciences naturelles et en génie du Canada, Ministères des Forêts, de la Faune et des Parcs, Observatoire régional de recherche en forêt boréale, Syndicat des producteurs des bois du SLSJ, Producteurs et productrices acéricoles du Québec, Centre ACER, and Fondation de l’Université du Québec à Chicoutimi.
References
Gscholar
Gscholar
Gscholar
Gscholar
Gscholar
CrossRef | Gscholar
Gscholar
Authors’ Info
Authors’ Affiliation
Gaurisha Bhatt 0009-0002-2187-8835
Gian De Lima Santos 0000-0001-7598-7389
Roberto Silvestro 0000-0003-2739-1534
Sergio Rossi 0000-0002-9919-0494
Laboratoire sur les Écosystèmes Terrestres Boréaux, Département des Sciences Fondamentales, Université du Québec à Chicoutimi, 555 boulevard de l’Université, Chicoutimi, G7H2B1, QC (Canada)
Department of Natural Sciences, Université du Québec en Outaouais - UQO, 58 Main Street, Ripon, QC J0V1W0 (Canada)
Corresponding author
Paper Info
Citation
Kurokawa SYS, Bhatt G, De Lima Santos G, Silvestro R, Delagrange S, Rossi S (2024). Measuring natural sap production in sugar maple at daily temporal resolution. iForest 17: 323-330. - doi: 10.3832/ifor4591-017
Academic Editor
Paola Cetera
Paper history
Received: Feb 20, 2024
Accepted: Aug 26, 2024
First online: Oct 19, 2024
Publication Date: Oct 31, 2024
Publication Time: 1.80 months
Copyright Information
© SISEF - The Italian Society of Silviculture and Forest Ecology 2024
Open Access
This article is distributed under the terms of the Creative Commons Attribution-Non Commercial 4.0 International (https://creativecommons.org/licenses/by-nc/4.0/), which permits unrestricted use, distribution, and reproduction in any medium, provided you give appropriate credit to the original author(s) and the source, provide a link to the Creative Commons license, and indicate if changes were made.
Web Metrics
Breakdown by View Type
Article Usage
Total Article Views: 4962
(from publication date up to now)
Breakdown by View Type
HTML Page Views: 3389
Abstract Page Views: 610
PDF Downloads: 859
Citation/Reference Downloads: 3
XML Downloads: 101
Web Metrics
Days since publication: 266
Overall contacts: 4962
Avg. contacts per week: 130.58
Article Citations
Article citations are based on data periodically collected from the Clarivate Web of Science web site
(last update: Mar 2025)
Total number of cites (since 2024): 2
Average cites per year: 1.00
Publication Metrics
by Dimensions ©
Articles citing this article
List of the papers citing this article based on CrossRef Cited-by.
Related Contents
iForest Similar Articles
Research Articles
Links between phenology and ecophysiology in a European beech forest
vol. 8, pp. 438-447 (online: 15 December 2014)
Research Articles
A resource capture efficiency index to compare differences in early growth of four tree species in northern England
vol. 10, pp. 397-405 (online: 24 March 2017)
Short Communications
Effect of intensive planting density on tree growth, wood density and fiber properties of maple (Acer velutinum Boiss.)
vol. 9, pp. 325-329 (online: 22 October 2015)
Research Articles
Inter- and intra-annual patterns of seed rain in the black spruce stands of Quebec, Canada
vol. 10, pp. 189-195 (online: 13 December 2016)
Research Articles
Wintertime photosynthesis and spring recovery of Ilex aquifolium L.
vol. 12, pp. 389-396 (online: 31 July 2019)
Research Articles
Soil nutrient status, nutrient return and retranslocation in poplar species and clones in northern Iran
vol. 6, pp. 336-341 (online: 29 August 2013)
Research Articles
Evaluation of fast growing tree water use under different soil moisture regimes using wick lysimeters
vol. 6, pp. 190-200 (online: 08 May 2013)
Research Articles
A comparison of models for quantifying growth and standing carbon in UK Scots pine forests
vol. 8, pp. 596-605 (online: 02 February 2015)
Research Articles
Decline in commercial pine nut and kernel yield in Mediterranean stone pine (Pinus pinea L.) in Spain
vol. 13, pp. 251-260 (online: 03 July 2020)
Research Articles
A state-and-transition approach to alpine grasslands under abandonment
vol. 3, pp. 44-51 (online: 02 March 2010)
iForest Database Search
Search By Author
Search By Keyword
Google Scholar Search
Citing Articles
Search By Author
Search By Keywords
PubMed Search
Search By Author
Search By Keyword