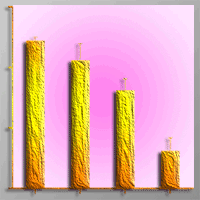
Can species Cedrela fissilis Vell. be used in sites contaminated with toxic aluminum and cadmium metals?
iForest - Biogeosciences and Forestry, Volume 14, Issue 6, Pages 508-516 (2021)
doi: https://doi.org/10.3832/ifor3890-014
Published: Nov 11, 2021 - Copyright © 2021 SISEF
Research Articles
Abstract
Toxic metals are among the main pollutants contributing to environmental degradation. Cadmium (Cd) and aluminum (Al) stand out among these metals as extremely toxic elements. The use of native species in reforestation programs can compensate for degradation and re-establish the ecological conditions of the affected environments. Cedrela fissilis Vell., popularly known as cedar, may be used as an alternative in phytoremediation, since it is a fast-growing native woody species widely distributed in tropical America. In this study we investigated the possibility of using C. fissilis in sites contaminated with Al and Cd by evaluating morphological, physiological, and biochemical variables of seedlings grown in hydroponic system. C. fissilis seedlings were subdivided into two experiments with a completely randomized design. The first experiment evaluated the effect of four Al concentrations, namely: 0 (complete nutrient solution without phosphorus), 25, 50 and 100 mg l-1. The second experiment evaluated four Cd concentrations, namely: 0 (complete nutrient solution), 25, 50 and 100 μM. Each sample unit consisted in a pot with four plants. Morphological, physiological and biochemical variables of seedlings were evaluated after 15-day exposure to different treatments in the hydroponic system. Aluminum concentration of 100 mg l-1 caused oxidative stress in C. fissilis seedlings, reduced shoot and root dry weight, and increased hydrogen peroxide contents, which led to lipid peroxidation. Cadmium concentration of 100 µM also damaged C. fissilis seedlings by significantly reducing root dry weight and involving the most severe effects on photosynthetic variables. Cadmium presence in the nutrient solution negatively affected morphophysiological and biochemical variables of Cedrela fissilis seedlings, and it was also harmful to their growth. Based on our results, the investigated species shows a sensitive behavior upon exposure to cadmium. On the other hand, C. fissilis tolerates high Al concentrations (up to 50 mg l-1), which suggests a moderate tolerance to this metal.
Keywords
Phytoremediation, Heavy Metals, Gas Exchange, Morphophysiological Variables
Introduction
Toxic metals associated with industrial and agricultural expansion stand out among the main abiotic agents capable of causing stress in living organisms. The bioaccumulation of these metals can be found at different environmental levels ([22]). In particular, metals such as cadmium (Cd) and aluminum (Al) are potentially toxic to different organisms even at low concentrations ([7]).
Cadmium is widespread in different environments and is extremely toxic to plants and animals ([17]). It is mainly released into the environment through human activities such as mining, metallurgy, cement factories, waste combustion and use of pesticides and fertilizers comprising Cd ([21]). Although Cd is not essential for plant nutrition, it can be easily absorbed by roots and accumulated in all plant tissues, from roots to shoot. Average Cd rate in Brazilian soils seen as uncontaminated is 0.18 mg Kg-1, whereas contaminated soils worldwide present Cd content ranging from 5.9 to 531 mg Kg-1, depending on the cause of pollution ([24]).
The main Cd-induced toxicity symptoms observed in plants comprise stunted growth, chlorosis, leaf epinasty, abnormal chloroplast ultrastructure, photosynthesis inhibition, enzyme inactivation in CO2 fixation processes, lipid peroxidation, disturbance in nitrogen and sulfur homeostasis, biological membrane oxidation and reduced root and stem growth, and concomitant reduction in biomass production ([1]).
Aluminum is one of the most abundant metals on Earth’s crust ([48]). Its toxicity is one of the biggest limitations to plant yield in acidic soils in tropical and subtropical sites worldwide ([7]). Brazilian soils are mostly weathered and acidic due to high Al and manganese (Mn) contents, as well as to low sum and saturation of bases ([6]). These soils show pH<5.0; under these conditions, Al is ionized to form phytotoxic ions (Al3+) that are promptly absorbed by plant roots, thus inhibiting root elongation and reducing crop yield ([16]). Since amelioration processes are hard to accomplish and highly expensive, soil acidity has become a major issue in subsurface soils; therefore, it is necessary to find crop plant species capable of tolerating acidic soils or high Al accumulation rates.
Root growth inhibition - which may occur after brief plant exposure to Al - is the first symptom of Al toxicity. Root meristems are considered the plant part most sensitive to Al toxicity, suggesting that Al actively interacts in cell division and elongation processes ([4]). Plants’ response to exposure to Al are associated with changes in physiological and biochemical processes, such as increased reactive oxygen species (ROS) production and damage to biological membranes. In addition, Al has negative effects on photosynthetic activity, such as decreased photosynthetic pigments and fluorescence parameters, reduced enzyme activity and carbohydrate metabolism, decreased stomatal conductance, and programmed cell death induction ([40]).
Given the harmful effect of these metals on plant components, it is essential to identify species capable of tolerating such contamination or accumulating these metals though time in order to revegetate and/or even decontaminate contaminated sites ([41]). Also, it is necessary understanding the mechanisms developed by tolerant and resistant plants to support the selection of species suitable to be used in sites contaminated with toxic metals.
Cedrela fissilis Vell. (cedar) stands out among several species with potential to recover degraded areas ([33]). This native woody species grows fast and is widely distributed in tropical America. C. fissilis has light and soft timber, which can be easily used for several purposes, such as plywood, moldings, sculptures, civil construction and as ornamental species ([14]). In addition, it presents high biomass production and filtering capacity, due to its extensive root system, which are features highly desirable for phytoremediation programs ([5]).
The aim of the present study is to investigate the suitability of C. fissilis to be used in remediation of areas contaminated with Al and Cd, by evaluating morphological, physiological and biochemical variables in hydroponic system.
Material and methods
Study site
The study was conducted in a greenhouse at the Biology Department of Federal University of Santa Maria (UFSM), Santa Maria Campus, RS, Brazil, under controlled temperature of approximately 25 °C, and mean humidity of 60%. Analyses were carried out at the Plant Physiology and Nutrition Laboratory of the Biology Department.
Experimental design
Cedrela fissilis seedlings were subdivided into two experiments, which followed a completely randomized design. The first experiment evaluated the effect of four aluminum (Al) concentrations: 0 (complete nutrient solution without phosphorus), 25, 50 and 100 mg L-1. Control treatment had nutritive solution without phosphorus (P) to avoid physical-chemical interactions between P and Al. The second experiment evaluated four cadmium (Cd) concentrations: 0 (complete nutrient solution), 25, 50 and 100 μM. Each sample unit consisted in a pot with four plants. The above Cd and Al concentrations were selected based on the scientific literature and on studies conducted by our research group with other plant species.
Seeds purchased in the UFSM Forest Nursery, Santa Maria Campus, were used to produce C. fissilis seedlings. They were sown in Carolina Soil® commercial substrate composed of Sphagnum sp. and vermiculite. Plastic trays (38 × 56 cm) were used as containers for seedling germination and initial growth. Substrate humidity was kept close to 60% of the field capacity and monitored on a daily basis throughout the experimental period.
Seedlings were irrigated with complete nutrient solution at pH 4.5 ± 0.1 on a weekly basis, from the 15th day after sowing (DAS) onwards. Nutrient solution was formed by 6090.5 μM of nitrogen (N); 974.3 μM of magnesium (Mg); 4986.76 μM of chlorine (Cl); 2679.2 μM of potassium (K); 2436.2 μM of calcium (Ca); 359.9 μM of sulfur (S); 243.592 μM of phosphorus (P); 0.47 μM of copper (Cu); 2.00 μM of manganese (Mn); 1.99 μM of zinc (Zn); 0.17 μM of nickel (Ni); 24.97 μM of boron (B); 0.52 μM of molybdenum (Mo) and 47.99 μM of iron (as FeSO4/Na-EDTA - [20]).
Seventy-three days after sowing, when seedlings were approximately 10 cm tall, they were carefully removed from the substrate in the trays and transferred to hydroponic system. Each seedling was placed in a 6-L pot filled with Hoagland & Arnon ([20]) complete nutrient solution. Polystyrene blade with four central holes was added to the surface of each pot to allow plants to pass through it. The polystyrene blade allowed plants to be fixed and reduced the solution evaporation from each pot.
Seedlings were left to acclimate for seven days in Hoagland’s nutrient solution, at 100% of its original concentration. Nutrient solution in each pot was aerated through PVC microtubes connected to air compressor. Its original form comprised the following concentrations (in mg L-1): NO3- = 196; NH4 = 14; P = 31; K = 234; Ca = 160; Mg = 48.6; S = 70; Fe-EDTA = 5; Cu = 0.02; Zn = 0.15; Mn = 0.5; B = 0.5; Mo = 0.01.
After the acclimation period, seedlings were subjected to treatments based on different Al and Cd availability conditions for 15 days; they remained in hydroponic system for 22 days, in total. Plants were collected as soon as metal toxicity symptoms were observed, mainly at the highest Al and Cd concentrations. Nutrient solution in each pot was replaced twice a week and its pH was adjusted on a daily basis to 4.5 ± 0.1 using 1.0 mol L-1 HCl or 1.0 mol L-1 NaOH.
Growth variables
One plant from each experimental unit was collected for growth assessment purposes. Shoot height and root length of each seedling were measured by a millimeter ruler. Measurements were taken before and after treatment application, and plant growth derived from the increase recorded within that period.
Seedlings were harvested and separated into shoots and roots, washed in running water and dried in forced air circulation oven at approximately 65 °C, until they reached constant weight. Shoot (SDW, g plant-1) and root dry weight (RDW, g plant-1) were determined.
Roots were subjected to morphological characterization based on digitized images using the software WinRhizo™ Pro 2013 (Regent Instruments Inc., Sainte-Foy, Quebec, Canada) coupled to a scanner Expression 11000® (EPSON Corp., Nagano, Japan) equipped with additional light (TPU) at 600 DPI resolution. Root length (cm plant-1), mean root diameter (mm) and number of branches were measured.
Photosynthetic variables
The third fully expanded leaf of each seedling was used to evaluate photosynthetic variables in an infrared gas analyzer (model Li-COR® 6400 XT, Lincoln, NE, USA) at 500 μmol photosynthetic radiation-2 s-1 and CO2 concentration of 400 μmol mol-1. Measurements were carried out in the morning between 8:00 and 10:00 am, before plants were collected for growth analysis. The following variables were determined: net CO2 assimilation rate (A), transpiratory rate (E), stomatal conductance (Gs), intercellular CO2 concentration (Ci), Rubisco’s instant carboxylation efficiency (A/Ci, based on the ratio between photosynthetic rate and intercellular CO2 concentration), and water use efficiency (WUE, based on the ratio between photosynthetic and transpiration rates).
Biochemical variables
Twelve plants from each treatment were collected and subjected to biochemical variable analysis, totaling 48 plants per experiment. Seedlings were separated into shoot and roots, washed in distilled water, placed in aluminum foil envelopes, and frozen with liquid nitrogen to avoid sample degradation. They were kept in ultra-freezer at -80 °C until they were pre-prepared for analysis. Sample preparation was carried out through manual maceration with liquid nitrogen until a fine powder was obtained for each sample. Subsequently, the specific amount of powder necessary for each analysis was weighted using a precision digital scale: 0.05 g of fresh sample was used for determining leaf pigments, 0.5 g for antioxidant enzymes, 0.3 g for hydrogen peroxide, and 0.5 g for lipid peroxidation.
Total chlorophylls and carotenoids content
The Hiscox & Israelstam ([19]) method was used for total chlorophyll and carotenoid extraction, whereas the Lichtenthaler ([26]) equation was used for their estimates. Previously weighed samples were added with 5 ml of dimethylsulfoxide (DMSO). Tubes were incubated at 65 °C for approximately 90 min, until full pigment release in a dark green solution was achieved. Subsequently, this solution was separated into two repetitions of 2 ml each. Solution absorbance was measured in UV-visible spectrophotometer (model 1105, Bel Photonics, Piracicaba, Brazil), at wavelength of 663, 645 and 470 nm for chlorophyll a, chlorophyll b and carotenoids, respectively. Total chlorophyll values corresponded to the sum of chlorophylls a and b.
Antioxidant enzyme activity
Antioxidant enzymes were determined by adding 0.5 g of sample to 3 mL of 0.05 M homogenization extraction buffer (pH 7.8) comprising 1 mM EDTA and 2% (w/v) polyvinylpyrrolidone (PVP). Homogenate was centrifuged in a high-speed refrigerated centrifuge (model CR22 N, Eppendorf Himac Technologies Co., Hitachinaka, Japan) at 13.000×g at 4 °C for 20 minutes. Supernatant was used to determine enzyme activity and protein concentration ([49]).
Guaiacol peroxidase (POD) enzyme activity was determined according to Zeraik et al. ([44]); guaiacol was used as substrate. Reaction mixture comprised 1.0 ml of potassium phosphate buffer (100 mM, pH 6.5), 1.0 ml of guaiacol (15 mM) and 1.0 ml of H2O2 (3 mM) in quartz cuvette. When the homogenization was complete, 50 µL of plant extract was added to the solution. Enzyme activity was measured through guaiacol oxidation into tetraguaiacol by increasing absorbance at 470 nm at 15-second reading intervals. Results were expressed in enzyme units per mg of protein (U mg-1 protein). The molar extinction coefficient of 26.6 mM-1 cm-1 was used for calculation purposes.
Superoxide dismutase (SOD) activity was determined based on the spectrophotometric method described by Giannopolitis & Ries ([13]). Reaction mixture (MIX), which was kept in the dark, comprised 50 mM of potassium phosphate buffer (pH 7.8), 13 mM of methionine, 0.1 mM of EDTA, 75 μM of nitrobluetetrazolium (NBT) and 2 μM of riboflavin. Photochemical production of blue formazan from NBT was monitored by increasing absorbance at 560 nm. Reaction process was carried out at 25 °C in test tubes (13 × 100 mm) filled with 2.8 ml of reaction mixture (MIX) and 200 μl of enzymatic extract from the respective samples. After pipetting, tubes were placed in the reaction chamber under 15 W fluorescent lamp. Reaction started when the light was turned on and stopped 2 minutes later, when the light was turned off; samples were read in UV-visible spectrophotometer. SOD unit was defined as the number of enzymes inhibiting NBT photoreduction by 50% ([2]). During the test, photochemically excited riboflavin was reduced by methionine in semiquinone, which donated an electron to oxygen and formed the superoxide radical, which in turn converted NBT into blue formazan. Superoxide dismutase has catalyzed this reaction.
Hydrogen peroxide content
Hydrogen peroxide content was determined according to Loreto & Velikova ([27]). Root and leaf samples were homogenized in 3.0 mL of 0.1% trichloroacetic acid (TCA). Homogenized samples were centrifuged, 0.5 ml of the supernatant was added to 0.5 ml of potassium phosphate buffer (10 mM) and 1 ml of KI (1 M), and samples were absorbed in spectrophotometer at 390 nm. H2O2 concentration in the supernatant was evaluated by comparing its readings to a standard calibration curve. H2O2 concentration was expressed as μmol g-1 fresh weight.
Membrane lipid peroxidation
Lipid peroxidation was determined based on malondialdehyde (MDA) concentration, based on the method by El-Moshaty et al. ([11]). Leaf and root samples were homogenized in 4.0 mL of sodium citrate buffer (pH 6.5) and centrifuged. In total, 1 ml of supernatant was added to 1 ml of 20% (w/v) trichloroacetic acid (TCA) added with 0.5% (w/v) thiobarbituric acid (TBA). The mix was heated to 95 °C for 40 min and cooled in ice bath for 15 min. Subsequently, it was centrifuged at 10.000×g for 15 minutes. Supernatant absorbance was read at 532 and 600 nm (to correct non-specific turbidity). Lipid peroxidation was expressed as nmol of MDA mg-1 protein.
Statistical analysis
Results were subjected to analysis of variance, based on the factorial model (factorial ANOVA). Significant differences were checked by F-test and treatment means compared by Tukey test (α = 0.05) using the Sisvar statistical software ([12]).
Results
Cadmium effects on Cedrela fissilis
ANOVA results showed significant differences (p≤0.05) among Cd treatments (different cadmium concentrations) for most growth-related morphological variables in C. fissilis seedlings reared in a hydroponic system. Cd concentrations of 50 and 100 µM promoted a significant reduction in root length increment (ILR - Fig. 1A), while seedlings grown at Cd concentration of 25 µM did not show difference in ILR in comparison to control (0 µM Cd). On the other hand, C. fissilis seedlings did not show significant difference in increment in shoots (IS) regardless of the tested concentrations (data not shown).
Fig. 1 - Mean values recorded for (A) largest root length increment (ILR), (B) root dry weight (RDW), (C) shoot dry weight (SDW), (D) root length, (E) root diameter and (F) number of branches in C. fissilis seedlings subjected to different Cd concentrations. Bars represent the mean ± standard deviation. Different lowercase letters indicate significant (p≤0.05) differences between treatment means after Tukey test.
Cadmium concentrations of 25, 50 and 100 µM had negative effects on shoot dry weight (SDW - Fig. 1C) and root dry weight (RDW - Fig. 1B), in comparison to the control treatment. Seedlings exposed to 100 µM of Cd had the shortest mean root length (Fig. 1D), as well as the smallest diameter (Fig. 1E) and number of branches (Fig. 1F). However, there was no significant difference in root volume at all tested Cd concentrations (data not shown).
Different Cd concentrations had significant effect (p ≤ 0.05) on most physiological variables analyzed in the present study. Net CO2 assimilation rate (A - Fig. 2A), stomatal conductance (Gs - Fig. 2B) and transpiration rate (E - Fig. 2C) showed significant decrease as Cd concentrations in the nutrient solution increased. The highest Cd concentration treatment (100 µM) had the most negative effects on the aforementioned parameters.
Fig. 2 - Mean values recorded for (A) net photosynthetic rate, (B) stomatal conductance (Gs), (C) transpiration, (D) intercellular CO2 concentration (Ci), (E) total chlorophyll (total Chl) and (F) carotenoids in C. fissilis seedlings subjected to different Cd concentrations. Bars represent the mean ± standard deviation. Different lowercase letters indicate significant (p≤0.05) differences between treatment means after Tukey test.
Cadmium concentration of 100 µM had the most significant effect on internal CO2 concentration (Ci - Fig. 2D). Cadmium levels of 0 and 25 µM were statistically similar in terms of both internal CO2 concentration (Fig. 2 D) and water use efficiency (WUE - data not shown). On the other hand, Cd treatments at 50 and 100 µM recorded the highest mean WUE in comparison to other treatments (data not shown). However, there was no significant difference between treatments on Rubisco carboxylation efficiency (A/Ci - data not shown), regardless of the tested concentrations.
Different Cd concentrations had a significant effect (p ≤ 0.05) on most biochemical variables analyzed in this study. Total chlorophyll increased at Cd concentration of 50µM (Fig. 2E), whereas an increased content in carotenoids was found at Cd concentrations of 25 and 50 µM (Fig. 2F).
A significant increase in the activity of superoxide dismutase (SOD) in the shoot of C. fissilis seedlings was detected when Cd concentration in the nutrient solution increased (Tab. 1). SOD activity reached the highest values at Cd concentrations of 50 and 100 µM, i.e., an increase by 37% in shoot at 100 µM. SOD activity increase by 10.83% was observed in the roots at Cd concentration of 100 µM, in comparison to the control treatment (Tab. 1).
Tab. 1 - Mean values recorded for superoxide dismutase (SOD) enzyme activity, guaiacol peroxidase enzyme (POD) activity, hydrogen peroxide content, and membrane lipid peroxidation in the shoot and roots of C. fissilis seedlings subjected to different Cd concentrations. Data represent the mean ± standard deviation. Different letters in rows indicate significant (p≤0.05) differences between treatment means after Tukey test.
Variable | Compartment | Treatment (Cd concentration) | |||
---|---|---|---|---|---|
0 µM | 25 µM | 50 µM | 100 µM | ||
SOD activity (U mg-1 protein) |
Shoot | 327.2 ± 4.5 c | 351.6 ± 18 bc | 418.3 ± 23.0 b | 524.5 ± 6.4 a |
Root | 338.3 ± 27 b | 322.7 ± 20.1 b | 315.7 ± 17.7 b | 379.3 ± 23.7 a | |
POD activity (U mg-1 protein) |
Shoot | 80.3 ± 10.3 c | 177.8 ± 5.6 b | 253.4 ± 7.6 a | 235.9 ± 10.5 a |
Root | 1358.6 ± 10.8 a | 774.7 ± 23.6 b | 736.4 ± 32.9 b | 510.3 ± 0.6 c | |
H2O2 (μmol g-1 FW) |
Shoot | 0.86 ± 0.02 b | 0.91 ± 0.02 b | 1.0 ± 0.01 a | 1.04 ± 0.05 a |
Root | 0.17 ± 0.02 a | 0.18 ± 0.01 a | 0.13 ± 0.01 b | 0.0 ± 0.01 c | |
MDA (nmol mg-1 protein) |
Shoot | 0.05 ± 0.01 c | 0.10 ± 0.02 a | 0.07 ± 0.0 b | 0.07 ± 0.0 b |
Root | 0.03 ± 0 c | 0.08 ± 0.001 a | 0.05 ± 0.0 bc | 0.05 ± 0.0 b |
The highest values recorded for guaiacol peroxidase (POD) activity in the shoots were observed in seedlings subjected to Cd concentrations of 25, 50 and 100 µM, which were significantly different from those recorded in control plants (Tab. 1). Contrastingly, a significant reduction in POD activity in roots was observed at the same Cd concentrations (25, 50 and 100 µM - Tab. 1).
A significant increase in hydrogen peroxide (H2O2) content in the shoot was detected at Cd concentrations of 50 and 100 µM (Fig. 2A). On the other hand, H2O2 content in the shoot and roots were similar (p>0.05) at Cd concentrations of 25 and 0 µM. However, there was negative correlation between H2O2 content and Cd concentration in the roots (Tab. 1), since H2O2 content decreased at Cd concentrations of 50 and 100 µM, in comparison to the control seedlings.
Lipid peroxidation values in the shoot at Cd concentrations of 25, 50 and 100 µM were higher than those recorded in the control plants (Tab. 1). On the other hand, lipid peroxidation in the roots at Cd concentrations of 25 and 100 µM was higher when compared with that in control seedlings (Tab. 1).
Aluminum effects on Cedrela fissilis
Analysis of variance revealed significant differences (p≤0.05) among different Al treatments (different Al concentrations) on most growth-related morphological variables.
The ILR (increment of the largest root) variable showed the lowest mean (15.93 cm) at Al concentration of 100 mg L-1, which was significantly (p<0.05) different from that recorded for control seedlings (42.97 cm - Fig. 3A). Al concentration of 100 mg L-1 reduced ILR by 37.07%, in comparison to the control treatment (Fig. 3A). In addition, seedlings grown at Al concentrations of 25 and 50 mg l-1 showed a significant ILR reduction. On the other hand, we found no significant differences in height growth (SI) of C. fissilis seedlings, regardless of Al concentrations (data not shown).
Fig. 3 - Mean values recorded for (A) largest root length increment (ILR), (B) root dry weight (RDW), (C) shoot dry weight (SDW), (D) root length, (E) root diameter, and (F) number of branches in C. fissilis seedlings subjected to different Al concentrations. Bars represent the mean ± standard deviation. Different lowercase letters indicate significant (p≤0.05) differences between treatment means after Tukey test.
Shoot dry weight (SDW - Fig. 3C) and root dry weight (RDW - Fig. 3B) also had the lowest means at the highest Al concentration (100 mg l-1), in comparison to the control treatment. However, SDW and RDW means at Al concentrations of 25 and 50 mg l-1 did not differ from those recorded for the control treatment (Fig. 3).
The application of different Al concentrations had the most severe effects on seedling root length (Fig. 3D), root diameter (Fig. 3E) and number of branches (Fig. 3F), in comparison to the control treatment. The highest means recorded for the above variables were observed in the control treatment (Fig. 3). Only the highest Al concentration (100 mg l-1 of Al) had negative effect on root volume.
There was significant effect (p≤0.05) of different Al concentrations on most photosynthetic variables analyzed in the present study. Aluminum concentrations of 25, 50 and 100 mg l-1 negatively affected net CO2 assimilation rate (A - Fig. 4A), stomatal conductance (Gs - Fig. 4B), and transpiratory rate (E - Fig. 4C) in C. fissilis seedlings in comparison to the control treatment. The highest means for these variables were recorded in the control treatment. The lowest mean internal CO2 concentrations (Ci) were recorded at Al concentrations of 50 and 100 mg l-1 (89.858 and 104.200 μmol CO2 m-2 s-1, respectively - Fig. 4D). All tested Al concentrations promoted a significant increase in water use efficiency (WUE - data not shown) in comparison with the control. On the other hand, there was no significant effect between treatments on Rubisco carboxylation efficiency (A/Ci - data not shown).
Fig. 4 - Mean values recorded for (A) net photosynthetic rate, (B) stomatal conductance (Gs), (C) transpiration, (D) intercellular CO2 concentration (Ci), (E) total chlorophyll (total Chl), and (F) carotenoids in C. fissilis seedlings subjected to different Al concentrations. Bars represent the mean ± standard deviation. Different lowercase letters indicate significant (p≤0.05) differences between treatment means after Tukey test.
Significant effects (p ≤ 0.05) of different Al concentrations on biochemical variables assessed were also observed. Indeed, 50 mg l-1 of Al promoted significant increase in total chlorophyll (Fig. 4E) and carotenoid contents in comparison to the control (Fig. 4F). Superoxide dismutase (SOD) activity in the shoot and roots of seedlings showed a significant increase at all tested Al concentrations (Tab. 2).
Tab. 2 - Mean values recorded for superoxide dismutase (SOD) enzyme activity, guaiacol peroxidase enzyme (POD) activity, hydrogen peroxide content , and membrane lipid peroxidation in the shoot and roots of C. fissilis seedlings subjected to different Al concentrations. Data represent the mean ± standard deviation. Different letters in rows indicate significant (p≤0.05) differences between treatment means after Tukey test.
Variable | Compartment | Treatment (Al concentration) | |||
---|---|---|---|---|---|
0 mg l-1 | 25 mg l-1 | 50 mg l-1 | 100 mg l-1 | ||
SOD activity (U mg-1 protein) |
Shoot | 365.5 ± 4.5 c | 500.91 ± 7.2 b | 522.1 ± 6.0 b | 1180.5 ± 9.6 a |
Root | 267 ± 4.8 c | 342.56 ± 2.8 b | 388.5 ± 1.6 b | 549.1 ± 3.1 a | |
POD activity (U mg-1 protein) |
Shoot | 245.7 ± 9.7 a | 192.22 ± 18 b | 148.8 ± 7.7 c | 143.8 ± 11 c |
Root | 1090 ± 8.3 a | 1202 ± 8.7 a | 1207 ± 12.6 a | 1203 ± 20 a | |
H2O2 (μmol g-1 FW) |
Shoot | 0.55 ± 0.02 c | 0.87 ± 0.01 b | 1.10 ± 0.06 a | 0.87 ± 0.04 b |
Root | 0.07 ± 0.00 c | 0.12 ± 0.01 b | 0.15 ± 0.00 b | 0.22 ± 0.02 a | |
MDA (nmol mg-1 protein) |
Shoot | 0.06 ± 0.00 bc | 0.04 ± 0.00 c | 0.07 ± 0.00 bc | 0.13 ± 0.00 a |
Root | 0.03 ± 0.00 b | 0.02 ± 0.00 b | 0.03 ± 0.00 b | 0.06 ± 0.00 a |
Lower guaiacol peroxidase (POD) activity was observed in the shoot of seedlings subjected to Al concentrations of 25, 50 and 100 mg l-1, in comparison to the control (Tab. 2). On the other hand, there was not significant difference in POD activity in the roots, regardless of the Al concentrations (Tab. 2).
The highest hydrogen peroxide (H2O2) content was observed in the shoot and in the roots of plants subjected to all tested Al concentrations (Tab. 2).
Lipid peroxidation in the shoot and roots was significantly increased in plants subjected to the highest Al concentration (100 mg l-1 - Tab. 2). On the other hand, lipid peroxidation in the shoot and roots of seedlings subjected to Al concentrations of 25 and 50 mg l-1 did not significantly differ from that of the control treatment (Tab. 2).
Discussion
Cadmium
Growth reduction in the shoot and roots is one of the main Cd toxicity symptoms in plants, leading to a decrease of biomass production in plants belonging to different species ([8]). However, shoot growth (SI - data not shown) was lesser affected than root growth in the present study (Fig. 1A) due to Cd addition to the nutrient solution. This could be attributed to the fact that roots are often in direct contact with contaminants ([21]), thus affecting the physiological response of plants to stress. Moreover, Cd is mostly retained in the roots and only smaller amounts are translocated to the shoots, whereby causing lesser damage to the latter organs ([17]).
Root increment inhibition (Fig. 1A) induced by Cd was observed at concentrations of 50 and 100 µM, corroborating the results reported by Ullah et al. ([37]) in Cicer arietinum L. cultivars subjected to high Cd stress (50 µM). This response can be attributed to cell cytoskeleton microtubule depolymerization and to chromosomal aberration formation, which lead to lower mitotic activity of meristematic cells ([18]).
Changes in root morphology may be part of integrated signal response to metal stressors. In fact, a stroFig. 1g reduction in the length, diameter and number of root branches (Fig. 1D-F) were observed under the highest Cd stress level (100 µM). These changes in root morphology under Cd stress may stem from changes in root development, such as reduced parenchymal cell and cortical tissue size, which decreased plants’ resistance to radial water flows.
Although different Cd concentrations did not affect shoot growth (data not shown), a reduced shoot dry weight was observed (Fig. 1C). This may be due to the reduced stem diameter or the decreased number of leaves, which resulted in lower shoot dry weight production from cedar seedlings (Fig. 1C). In addition, reduced root growth, along with changes in root morphological variables (Fig. 1D-F), have also negatively affected the biomass production of cedar seedling, since smaller roots absorb lesser water and nutrient amounts.
Plants subjected to stress conditions adjust their relative biomass allocation in their organs, in a process known as allocation plasticity. They also change basic processes such as photosynthesis and respiration ([9]). Photosynthetic ability in plants is mainly regulated by photochemical reactions, which enable energy production, gas exchange, as well as CO2 fixation and assimilation. However, Cd can damage plants’ photosynthetic ability, induce oxidative stress, inhibit stomatal opening and reduce nitrate and iron absorption ([42]).
In this study, significant reductions in CO2 assimilation rate (A - Fig. 2A), stomatal conductance (Gs - Fig. 2B), and transpiration rate (E - Fig. 2C) was observed as Cd concentrations in nutrient solution increased. The most severe effect of Cd on these variables was recorded at Cd concentration of 100 µM. Decreased net assimilation rate (Fig. 2A) may be associated with limited CO2 diffusion to the Rubisco carboxylation site, due to decreased stomatal conductance (Fig. 2B). Heavy metal-related stress can lead to decreased stomatal conductance, thus limiting the photosynthetic ability ([22]).
Cadmium decreases partial CO2 pressure in the stomata, which leads to stomatal closure and decreased transpiration. One of the harmful effects of Cd lies on calcium induction in the endoplasmic reticulum and vacuole. This process increases cation levels in the cytosol, which is associated with stomatal closure ([31]). Thus, Cd affects stomatal opening and closure, mainly due to increased leaf osmotic potential and to direct Cd action on guard cells. In addition, Gs reduction (Fig. 2B) may occur due to root growth inhibition, which limits water absorption and leads to stomatal closure. Moreover, high chemical element concentration leads to metabolic decline, leaf turgidity loss and hydropassive stomatal closure ([34]). Therefore, the observed reduction in transpiration rates is directly linked to the reduction in assimilation rate and stomatal conductance (Fig. 2).
The highest water use efficiency (WUE) values (data not shown) were found at Cd concentrations of 100 and 50 µM, as the stress caused by Cd forced plants to close their stomata. This decreased the stomatal conduction (Fig. 2B) and led to areduced transpiration rate (Fig. 2C), which in turn resulted in higher WUE.
The photosynthetic performance of seedlings is also affected by the pigments involved, such as total chlorophyll and carotenoids ([36]). The observed increase in total chlorophyll and carotenoid contents in plants exposed to Cd concentration of 50 µM (Fig. 2E-F) was not sufficient to counterbalance the reduction in photosynthetic performances due to Cd stress. This response may be associated with the fact that Cd stress impairs chloroplast structure and affects their stable binding to proteins, thus damaging the photosynthetic apparatus. In fact, Cd ions may change the activity center of enzymes and replace Mg2+ in the chlorophyll molecule ([45]), decreasing the light harvesting ability of chlorophyll and affecting the activity of enzymes associated with chlorophyll synthesis. This process has negative effects on photosynthetic variables, leading to the reduction of seedling dry weight ([45]). These inhibitory effects may also be associated with indirect Cd interaction with micronutrients, which act as cofactors for enzymes, pigments and structural components of the photosynthetic apparatus.
Carotenoid contents in seedlings subjected to 50 µM of Cd have increased to protect the photosynthetic apparatus and avoid singlet oxygen formation (reactive oxygen species - ROS). This occurs because carotenoids are non-enzymatic antioxidants acting as photoprotective pigments in the photosynthetic system by suppressing ROS and free radicals’ formation ([32]). Increased carotenoid content is the result of a natural strategic defense mechanism to combat the toxic effect of oxidative stress generated under Cd stress.
Plants are equipped with a natural defense system made by enzymatic and non-enzymatic antioxidants, which act as a protection from the oxidative damage induced by different environmental stress types ([17]). Indeed, ROS can be produced through different routes, such as the imbalance in electron transport chains of chloroplasts and mitochondria. Cadmium can lead to ROS generation by inducing chloroplast disturbance ([42]). Antioxidant enzymes such as superoxide dismutase (SOD) and guaiacol peroxidase (POD), play a key role in maintaining homeostasis in plant cells ([45]) by maintaining cellular redox status and prevent damage caused by ROS accumulation. In particular, SOD plays crucial role in removing the radical O2-•, whose decomposition is always followed by H2O2 production which acts as oxidizer and reducer ([38]). The results of this study showed that SOD activity in the shoot gradually increased as Cd concentrations in the nutrient solution also increased (Tab. 1), as a consequence of the activation of the existing enzyme stock by the increased free radical production ([17]). The increased SOD activity in the shoot (Tab. 1) indicated that free radicals resulting from stress were neutralized via the formation of H2O2. However, high H2O2 accumulation is extremely harmful to the cellular metabolism, and POD converts H2O2 into water and oxygen. POD plays an essential role in providing tolerance to plants exposed to unfavorable conditions ([25]). In this study we observed an increased POD activity in the shoot, which may due to the increase in H2O2 released by SOD activity as a consequence of Cd stress (Tab. 1). On the other hand, POD activity inhibition was detected in the roots of seedlings subjected to all tested Cd concentrations (Tab. 1). This may be associated with a decreased enzyme synthesis or with changes in the assembly of its subunits ([39]). Excessive metal accumulation can inhibit enzymes by binding to catalytic active groups or by causing protein denaturation. Thus, POD activity often decreases when stress exceeds plants’ regulatory capacity ([46]). The decrease in POD activity suggests a likely delay in ROS removal, which implies an increase in lipid peroxidation which contributes to seedling growth inhibition.
Nonetheless, the increased POD activity in the shoot, mainly at Cd concentrations of 50 and 100 µM, was unsuccessful in counteracting the H2O2 accumulation (Tab. 1), as H2O2 content in the shoot significantly increased as Cd concentrations increased in the nutrient solution ([21]). According to Weifeng et al. ([38]), the high H2O2 rates observed under Cd stress were likely accountable for lipid peroxidation, as indicated by the excessive malondialdehyde (MDA) accumulation. MDA is an oxidized product of membrane lipids; it accumulates in plants exposed to oxidative stress. Therefore, MDA concentration in plants is often seen as an indicator of lipid peroxidation, as well as of the level of oxidative stress ([21]). In this study, the highest MDA content observed in the shoot was found at Cd concentrations of 25, 50 and 100 µM (Tab. 1), while the highest MDA content in the seedling roots was recorded at Cd concentrations of 25 and 100 µM. Thus, our results indicate that different Cd concentrations can change the original balance of plasma membrane permeability, as well as increase ROS production and induce antioxidant protein expression as adaptive response to neutralize ROS excess and minimize damage.
Aluminum
Root growth in this study was significantly inhibited by aluminum in the nutrient solution, especially at concentrations of 50 and 100 mg l-1 (Fig. 3A). On the other hand, shoot growth was lesser affected by Al, regardless of the tested concentrations (data not shown). Indeed, roots are one of the organs mostly sensitive to Al, resulting in the inhibition of main axis elongation, as well as in the limited development of lateral roots, which leads to short and poorly-developed root system ([3], [47]).
In addition, different Al concentrations significantly reduced the length, diameter and number of branches in cedar seedling roots (Fig. 3D-F). This is likely due to the strong binding of Al to negatively charged carboxylic groups on roots’ cortical and epidermal cell walls, thus changing ions binding and distribution in the apoplast and directly affecting root growth ([10]). Also, changes in morphological characteristics of roots can be caused by apoplastic lesions and interactions in root plasma membrane ([48], [47]), which leads to deficient mineral nutrient and water acquisition ([15]).
Root growth inhibition and changes in root morphological variables negatively affected root and shoot biomass production in plants exposed to 100 mg l-1 of Al (Fig. 3). The aluminum translocation to the shoot may have negatively influenced the formation and growth of these organs, which implied reduced photosynthetic rate (Fig. 4A) and lower biomass production ([16]). Moreover, Al stress may be responsible for determining structural changes in plant tissues ([30]) and impairing the functioning of photosynthetic apparatus, as suggested by the low net photosynthetic rates (A - Fig. 4A), associated with the decrease in stomatal conductance (Gs - Fig. 4B) and internal CO2 concentration (Ci - Fig. 4D) observed in seedlings under Al stress.
Aluminum may change stomatal behavior and directly influence stomatal closure ([4]); consequently, it inhibits plant transpiration (E - Fig. 4C) and increases water use efficiency, especially at the highest Al concentration (100 mg l-1). A possible explanation is that higher Al concentrations in the nutrient solution enhances cuticle and epicuticular wax development and leads to higher WUE ([35]). In addition, seedlings exposed to Al showed reduced stomatal conductance, which may also result in higher WUE.
Photosynthetic pigments are extremely sensitive to stress caused by toxic metals and can be used as reliable markers of stress caused by these metals ([1]). Aluminum concentration of 50 mg l-1 led to significant increase in total chlorophyll content in comparison to the control (Fig. 4E). However, the increase in photosynthetic pigment contents did not hinder a decrease in photosynthesic rates and, consequently, in dry biomass production (Fig. 3B-C). Moreover, high concentrations of Al have been reported to damage thylakoid membranes and replace compounds in the chlorophyll metabolic pathway ([28]). Aluminum can also affect chlorophyll biosynthesis by inhibiting δ-aminolavulinic acid dehydratase activity, which accounts for chlorophyll molecule and cytochrome formation ([7]). Therefore, higher concentrations of this ion can change chlorophyll and carotenoid composition, which can limit plant metabolic potential ([29]).
Al concentration of 50 mg l-1 has also promoted significant increase in carotenoid content in C. fissilis seedlings compared to control (Fig. 4F). Carotenoids play an essential role in protecting the photosynthetic apparatus against the harmful effects of light and oxygen by dissipating the excess of light in the form of heat in antenna pigment complexes ([32]). However, whenever carotenoids fail to dissipate ROS, antioxidant enzymes such as SOD and POD dismute ROS and maintain the cell homeostasis. In this study, we observed a gradual SOD activity increase in the shoot and roots as Al concentrations increased (Tab. 2). This suggests that plants’ ability to detoxify ROS was positively regulated ([47]). However, long-term Al stress can overcome the antioxidant capability of plant tissues. This results in ROS overproduction, which can damage biological membranes, have negative effects on photosynthetic activity, reduce enzyme activity and, finally, lead to programmed cell death ([45]).
The presence of aluminum at any concentration in the nutrient solution has significantly reduced POD activity in the shoot of cedar seedlings, but not in the roots (Tab. 2). Conversely, the increased SOD activity in seedling tissues indicated that free radicals were neutralized and detoxified as H2O2 (Tab. 2). This may explain the observed accumulation of H2O2, which is released as a product of SOD activity but not properly removed by POD activity in the shoot.
H2O2 also plays an important role in signal transduction during plant abiotic stress, since it acts as biomarker of tissue damage induced by ROS overproduction ([43]). However, excessive H2O2 accumulation can be extremely harmful, leading to lipid peroxidation and increased plasma membrane permeability. Indeed, Al can bind to phospholipids and/or change the fatty acid composition in the plasma membrane, reducing its fluidity and increasing its permeability, and ultimately leading to lipid peroxidation ([3]).
Lipid peroxidation in the shoot and roots has significantly increased at the highest Al concentration (100 mg l-1 - Tab. 2). On the other hand, MDA contents in the roots of seedlings subjected to Al concentrations of 25 and 50 mg L-1 did not differ from that of control plants (Tab. 2). The higher MDA content observed at the highest Al concentration (100 mg L-1) can be the direct effect of Al toxicity, as well as indicate oxidative stress in cedar seedlings, which can cause irreversible damage to plant tissue development and function in the long run.
Our results demonstrate that Cedrela fissilis can tolerate moderate Al concentrations in the growth medium. Aluminum tolerance mechanisms involve the exclusion via root organic acid exudation, changes in carbohydrate components of cell walls capable of binding Al in roots, as well as the uptake and subsequent sequestration either in the root or leaf cell vacuoles, where it is detoxified and sequestered ([23]). Besides, symplasmic tolerance to internal Al is also achieved by complexing Al with several organic compounds such as citrate, malate, and oxalate, as well as by secreting or sequestering the resulting complexes in vacuoles through several membrane-localized transport proteins, or by activating enzymatic and non-enzymatic antioxidants.
Conclusion
The presence of cadmium in the nutrient solution has negatively affected morphophysiological and biochemical variables in Cedrela fissilis seedlings, hindering their growth. Therefore, the species is sensitive to the exposure to cadmium. On the other hand, it has tolerated high Al concentrations (up to 50 mg l-1), which may indicate its tolerance to this metal.
Acknowledgements
The authors are grateful to Coordenação de Aperfeiçoamento de Pessoal de Nível Superior (CAPES) and to Conselho Nacional de Desenvolvimento Científico e Tecnológico (CNPq) for providing financial support to the postgraduation programs, as well as to the Federal University of Santa Maria for providing the space and equipment for the current study.
Authors’ contributions
CCK, MVMA, GSWO, DB, MB and LAT conceived and designed the experiments and analyzed the data; CCK, MVMA and GSWO carried out the physiological and photosynthetic analyses; CCK, MVMA, GSWO, DB, MB and LAT carried out the biochemical analyses; CCK, MVMA and GSWO wrote the manuscript, and LAT revised it.
References
CrossRef | Gscholar
CrossRef | Gscholar
CrossRef | Gscholar
Gscholar
Gscholar
CrossRef | Gscholar
Authors’ Info
Authors’ Affiliation
Gerne Silva Wertonge de Oliveira 0000-0001-7695-3789
Marcos Vinicius Miranda de Aguilar 0000-0003-0480-6119
Daniele Bernardy 0000-0001-6058-3584
Mirian Berger 0000-0003-1094-5270
Luciane Almeri Tabaldi 0000-0002-3644-2543
Departamento de Biologia, Universidade Federal de Santa Maria, Av. Roraima 1000, Cidade Universitária Bairro - Camobi, Santa Maria-RS,97105-900 (Brasil)
Corresponding author
Paper Info
Citation
Castro Kuinchtner C, Silva Wertonge de Oliveira G, Miranda de Aguilar MV, Bernardy D, Berger M, Tabaldi LA (2021). Can species Cedrela fissilis Vell. be used in sites contaminated with toxic aluminum and cadmium metals?. iForest 14: 508-516. - doi: 10.3832/ifor3890-014
Academic Editor
Werther Guidi Nissim
Paper history
Received: Jun 01, 2021
Accepted: Aug 27, 2021
First online: Nov 11, 2021
Publication Date: Dec 31, 2021
Publication Time: 2.53 months
Copyright Information
© SISEF - The Italian Society of Silviculture and Forest Ecology 2021
Open Access
This article is distributed under the terms of the Creative Commons Attribution-Non Commercial 4.0 International (https://creativecommons.org/licenses/by-nc/4.0/), which permits unrestricted use, distribution, and reproduction in any medium, provided you give appropriate credit to the original author(s) and the source, provide a link to the Creative Commons license, and indicate if changes were made.
Web Metrics
Breakdown by View Type
Article Usage
Total Article Views: 29404
(from publication date up to now)
Breakdown by View Type
HTML Page Views: 25911
Abstract Page Views: 1619
PDF Downloads: 1372
Citation/Reference Downloads: 2
XML Downloads: 500
Web Metrics
Days since publication: 1330
Overall contacts: 29404
Avg. contacts per week: 154.76
Article Citations
Article citations are based on data periodically collected from the Clarivate Web of Science web site
(last update: Mar 2025)
(No citations were found up to date. Please come back later)
Publication Metrics
by Dimensions ©
Articles citing this article
List of the papers citing this article based on CrossRef Cited-by.
Related Contents
iForest Similar Articles
Review Papers
Heavy metals and woody plants - biotechnologies for phytoremediation
vol. 4, pp. 7-15 (online: 27 January 2011)
Review Papers
A review of the performance of woody and herbaceous ornamental plants for phytoremediation in urban areas
vol. 13, pp. 139-151 (online: 14 April 2020)
Research Articles
Bioaccumulation of long-term atmospheric heavy metal pollution within the Carpathian arch: monumental trees and their leaves memoir
vol. 17, pp. 370-377 (online: 27 November 2024)
Research Articles
Heavy metal (Zn, Pb, Cd) concentration in soil and moss (Pleurozium schreberii) in the Brynica district, southern Poland
vol. 4, pp. 176-180 (online: 11 August 2011)
Research Articles
Variation of major elements and heavy metals occurrence in hybrid aspen (Populus tremuloides Michx. × P. tremula L.) tree rings in marginal land
vol. 13, pp. 24-32 (online: 15 January 2020)
Research Articles
Temporal analysis of pollutant metals in trees of three parks in Mexico City’s Metropolitan Area
vol. 18, pp. 138-145 (online: 01 June 2025)
Research Articles
Analysis of biometric, physiological, and biochemical traits to evaluate the cadmium phytoremediation ability of eucalypt plants under hydroponics
vol. 10, pp. 416-421 (online: 24 March 2017)
Research Articles
Heavy metal accumulation characteristics of Nepalese alder (Alnus nepalensis) growing in a lead-zinc spoil heap, Yunnan, south-western China
vol. 7, pp. 204-208 (online: 27 February 2014)
Short Communications
A quick screening to assess the phytoextraction potential of cadmium and copper in Quercus pubescens plantlets
vol. 10, pp. 93-98 (online: 13 October 2016)
Research Articles
Preliminary results of the tolerance to inorganic contaminants and phytoextraction potential of twelve ornamental shrub species tested on an experimental contaminated site
vol. 11, pp. 442-448 (online: 18 June 2018)
iForest Database Search
Search By Author
- C Castro Kuinchtner
- G Silva Wertonge de Oliveira
- MV Miranda de Aguilar
- D Bernardy
- M Berger
- LA Tabaldi
Search By Keyword
Google Scholar Search
Citing Articles
Search By Author
- C Castro Kuinchtner
- G Silva Wertonge de Oliveira
- MV Miranda de Aguilar
- D Bernardy
- M Berger
- LA Tabaldi
Search By Keywords
PubMed Search
Search By Author
- C Castro Kuinchtner
- G Silva Wertonge de Oliveira
- MV Miranda de Aguilar
- D Bernardy
- M Berger
- LA Tabaldi
Search By Keyword