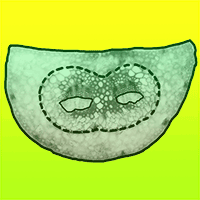
Long-term effects of stem girdling on needle structure in Scots pine
iForest - Biogeosciences and Forestry, Volume 11, Issue 4, Pages 476-481 (2018)
doi: https://doi.org/10.3832/ifor2648-011
Published: Jul 02, 2018 - Copyright © 2018 SISEF
Research Articles
Abstract
Stem girdling is the process of completely removing a strip of cork and phloem tissue. Phloem is the living tissue which serves as the main long-distance pathway for transporting carbohydrates produced during photosynthesis to all parts of the plant where needed, from source leaves to sinks. Stem girdling has been used to study several functional aspects of phloem and their direct impacts on tree growth. Although both photosynthesis and transpiration processes take place in needles, no studies exist which investigate the effect of source-sink disturbance on needle structure. In this study, we evaluated changes in needle morphology and anatomy in current-year Scots pine needles 227 and 411 days after girdling (DAG). Although the studied needle parameters recorded 227 DAG were from 2 to 20% higher than the same parameters in control needles, the differences were not significant. On the other hand, needles 411 DAG were thinner, with decreased cross-sectional areas, phloem areas, vascular cylinder areas, needle dry mass, needle density, and needle flatness when compared to control needles. Marked variations in needle growth were observed 411 DAG, with a smaller number of correlations among almost all studied needle parameters in needles 411 DAG when compared to control needles or needles 227 DAG. Structural development determining needle flatness, needle density, and leaf mass per area (LMA) appeared to have driving factors that were independent of the other studied needle parameters, as correlations with other parameters were not significant in any treatment. The changes in overall needle structure observed after long-term stem girdling provide new insights into the processes that occur as a result of source-sink disturbances. This type of data could be helpful, for example, in studies specifically focused on phloem transport, tree carbon relationships, or investigations modeling gas exchange. Our study might also support gene expression studies, which could provide further knowledge about the regulatory mechanisms that determine needle size and structural form.
Keywords
Anatomy, Cross-section, Morphology, Pinus sylvestris, Phloem, Source-Sink Disturbance
Introduction
Phloem serves as the long-distance pathway for transporting carbohydrates from source leaves to sink regions of active growth, storage structures, and other non-photosynthetic cells, including those in roots ([15]). Artificial girdling, i.e., the complete removal of a strip of bark and phloem around a tree’s outer circumference, has traditionally been used to study several functional aspects of phloem tissue and their impacts on tree growth as a transport pathway ([18]), on apical dominance ([35]), on the phloem-xylem connection ([38]), on processes involving bark regeneration ([20], [25]), and relationships between tree water and carbon status ([13], [6]). Phloem girdling has also been practiced in forestry as a pre-harvest treatment to alter wood properties by manipulating annual ring width, wood density, the duration of cambial activity, and latewood production ([24], [35], [8]). Despite its ecological significance, studies about the anatomical and physiological effects of stem girdling in forest trees are scarce compared to those carried out on branch girdling in horticulture ([15]).
The effects of stem girdling differ from those of branch girdling in that it promotes carbohydrate accumulation above the girdle and prevents carbohydrates from being transported to the roots ([15]). The first visual symptom after girdling is an increase in stem diameter above the girdle due to the growth of bark and xylem stimulated by the accumulation of carbohydrates, coupled with the interruption of stem growth below the girdle ([4], [7]). Roots are gradually depleted of their carbohydrate reserves and suffer starvation ([33]). Although the structural adaptation of stems to disruption in phloem long-distance transport has been studied in detail, to the best of our knowledge no research exists on the structural adaptation of leaves or needles in response to stem girdling. Only a handful of studies have focused on the development of leaf area and leaf mass per unit area (LMA) after girdling ([5], [8]) and only one study focused on needle xylem structure development after stem girdling ([11]). It was observed that long-term stem girdling led to a reduction in the theoretical hydraulic conductivity of Scots pine needles, and in their xylem area and number of tracheids ([11]). The physiological adaptation of leaves after girdling, on the other hand, has been studied more thoroughly. The accumulation of carbohydrates in leaves after girdling leads to the consequent down-regulation of photosynthetic rates ([28]), decreased stomatal conductance and transpiration ([29]), the accumulation of abscisic acid in leaves ([30]), and an increase in leaf water potential ([34]). It has also recently been shown that non-structural carbohydrates (NSC) regulate the gene expression involved in several plant metabolic functions ([12], [19]). Such a broad range of physiological adaptations in leaves after stem girdling should also trigger structural adaptation. In higher plants, the coordination of metabolism and morphogenesis often depends on hormone signals from one part of the plant to another ([31]). For example, the transport of auxin from the shoot to the root affects various developmental processes, including stem elongation and leaf senescence ([31]). Thus, the other interesting question is how the disruption of the phloem transport of plant hormones affects the interplay among the various highly correlated structural parameters of leaves. As both photosynthesis and transpiration processes take place in leaves, further knowledge about how leaves adapt to girdling is important for a better understanding of the physiology of the whole tree ([36]).
The aim of this study was to gain an insight into the anatomical and morphological responses of Scots pine (Pinus sylvestris L.) needles to the disturbance of phloem long-distance transport channels. We focused on needle structural development 8 and 14 months after girdling (hereafter referred to as 227 and 411 DAG, days after girdling, respectively). This study follows up on our previous work ([11]), in which needle xylem structure, sap flow, and non-structural carbohydrate (NSC) changes after stem girdling were analyzed. We hypothesize that (i) needle growth will increase in response to carbohydrate accumulation 227 DAG, (ii) needle growth will be reduced 411 DAG, as root starvation will lead to decreased sap flow and to a subsequent water shortage in the needles, and (iii) correlations among the studied needle morphological parameters will be different in girdled and control trees.
Material and methods
Study site and description of the experiment
The study site is an even-aged mixed coniferous/deciduous forest stand located near Brno (south-east of the Czech Republic - 49° 15′ 39″ N, 16° 36′ 20″ E; 340 m a.s.l.). The mean annual air temperature and precipitation are 8.7 °C and 490 mm, respectively. In 2014, the mean air temperature was 11.3 °C and the sum of precipitation was 609 mm. The stand was planted in 1928, with Scots pine (Pinus sylvestris L.) being the dominant tree species. The soil type is mesotrophic Cambisol. Twenty two co-dominant Scots pine trees were selected for detailed analysis. Six trees were selected as controls, 8 trees were stem girdled in summer, on 15 July 2013, and 8 trees were girdled in winter, on 15 January 2014. By the time of the summer girdling, the needles were fully developed in length. Stem cambium was active until September; however, most of the cells in the growth ring had already been formed at the time of summer girdling and a decline in the number of cells upon the enlargement phase suggested a transition from earlywood to latewood formation ([9]). Girdling consisted of removing a 7-10 cm wide band of bark (periderm, cortex, secondary phloem, and cambium) from around the entire stem at a height of 1.3 m above the ground. The girdled area was completely covered with resin at the end of the experiment. Sap flow was monitored in all experimental trees from 1 April to 30 August 2014, using EMS 51 sap flow meters (EMS Brno, Czech Republic). Current year needles from sun-exposed branches were also used to analyze levels of non-structural carbohydrates (NSC). A detailed description of girdling treatment, sap flow, and NSC analysis is given in Gebauer et al. ([11]).
Needle structure analysis
Five current-year shoots with fully developed needles were sampled from each of the trees. The shoots were obtained from branches at the middle height of the crown. The shoots were facing south and situated on the outer side of the crown. Samples were collected on 30 August 2014 (i.e., 227 or 411 days after girdling, hereafter referred to as DAG). In the field, the samples were fixed in FineFIX® solution (Milestone, Bergamo, Italy). Subsequently, from each of the collected shoots, four randomly selected fascicles (with two needles per fascicle, thus yielding 40 needles per tree) were collected for further needle structure analysis. One needle from each fascicle was used for cross-sectional anatomical analysis, while the second one was scanned immediately after removal from the fixative solution to determine the projected needle area (PLA) and individual needle length (Ln) using the software ImageJ® (National Institute of Mental Health, Bethesda, Maryland, USA). Scanned needles were then oven dried at 85 °C for 48 hours to determine needle dry mass (DM). The leaf mass per unit area (LMA) was calculated as the ratio of DM/PLA and the needle density (ND, g cm-3) was calculated according to eqn. 1:
Cross-sections for anatomical analyses were taken at the needle base and then examined under an Olympus BX51® light microscope (Olympus, Tokyo, Japan) at magnifications of up to ×400 and photographed using a Canon EOS® 1100D digital camera (Canon Inc., Tokyo, Japan) connected to a computer by means of QuickPhotoMicro 3.1 software (Promicra, Prague, Czech Republic). The following parameters were measured using ImageJ software (Fig. 1): cross-sectional needle area (An), vascular cylinder area (Avas), phloem area (Ap), needle thickness (D1), and needle width (D2). Needle cross-sectional shape (i.e., needle flatness, Fn) was calculated as the ratio of D1/D2. A needle with a round or square cross-section would have a value of Fn close to 1, and lower ratios would characterize more flattened needles.
Fig. 1 - Measured needle parameters: needle thickness (D1), needle width (D2), needle cross-sectional area (An, solid bold line), vascular cylinder area (Avas, dashed bold line), needle phloem area (Ap , solid fine line).
Statistical analysis
The linear mixed effect model (LME) employing the restricted maximum likelihood method (REML) was used, where individual trees were specified as a random effect ([37]). Where appropriate (i.e., for the parameters DM, Ln, LMA, ND, and PLA), the simple linear model was used. Linear models were also used for correlation matrices. Next, the Tukey’s honestly significance difference (HSD) test was used to indicate which treatments differed (significance level 0.05), and p-values assessing the probability of difference between treatments were extracted. The R statistical program ([27]) was used for statistical analysis. LME was fitted using the lme function from the nlme library, while Tukey’s test was performed using the glht function from the multcomp library. All acronyms, abbreviations, and symbols are defined in Tab. 1.
Tab. 1 - Overview of the needle parameters, their abbreviations, definitions and units used throughout this study.
Parameter | Explanation | Unit |
---|---|---|
An | needle cross-sectional area | mm2 |
Ap | needle phloem area | mm2 |
Avas | vascular cylinder area | mm2 |
D1 | needle thickness | mm |
D2 | needle width | mm |
DM | needle dry mass | mg |
Fn | needle flatness (D1/D2) | - |
Ln | needle length | mm |
LMA | leaf mass per area (DM/PLA) | g m-2 |
ND | needle density | g cm-3 |
PLA | needle projected area | mm2 |
Results
Needle structure
Although all studied needle structural parameters 227 DAG were slightly higher in girdled than in control trees (except no difference in Fn), these differences were not statistically significant (Fig. 2 and Tab. 2). Only LMA, which was approximately 9% higher in needles 227 DAG than in control needles, had a p-value close to the level of significance (p = 0.06). In contrast, nearly all studied needle parameters were significantly changed 411 DAG. On average, needles 411 DAG were shorter, thinner, denser, and lighter than control needles by 10, 32, 31, and 16%, respectively (Ln, D1, ND, DM, respectively - Fig. 2). Also, the An, Ap, Avas, and Fn of needles 411 DAG were lower by 26, 52, 36 and 30%, respectively, resulting in a substantial decrease in the cross-sectional dimensions of these needles compared to control needles (Fig. 2 and Tab. 2). Needles 411 DAG were flatter (Fn decrease) than control needles due to substantial decreases in D1, and not D2. Contrary to most of the other needle parameters 411 DAG, Ln was not significantly different from the same parameter in control needles (Tab. 2). Also, LMA and PLA values in needles 411 DAG and in control needles were similar (Tab. 2). Although LMA did not differ significantly between girdled and control trees, the LMA of needles 227 DAG was significantly higher than the LMA of needles 411 DAG (Tab. 2).
Fig. 2 - Means of morphological and anatomical parameters of Scots pine control needles, needles 227 days after girdling (DAG) and needles 411 DAG. Points in grey represent measured data. Vertical bars are 95% confidence intervals derived from the appropriate model. Parameters that are different at the 5% significance level are indicated by different letters. See Tab. 1 for an explanation of needle parameters.
Tab. 2 - P-values from Tukey’s HSD test showing the probability of differences in parameters between control needles and needles 227 or 411 days after girdling (DAG), and between needles 227 and 411 DAG. For an explanation and definition of the parameters, see Tab. 1.
Parameter | p-value | ||
---|---|---|---|
control × 227 DAG | control × 411 DAG | 227 DAG × 411 DAG | |
An | 0.50 | <0.001 | <0.001 |
Avas | 0.78 | <0.001 | <0.001 |
Ap | 0.70 | <0.001 | <0.001 |
D1 | 0.85 | <0.001 | <0.001 |
D2 | 0.70 | 0.99 | 0.74 |
DM | 0.37 | 0.003 | 0.07 |
Fn | 0.99 | <0.001 | <0.001 |
Ln | 0.94 | 0.64 | 0.43 |
LMA | 0.06 | 0.76 | 0.01 |
ND | 0.24 | <0.001 | 0.04 |
PLA | 0.68 | 0.54 | 0.15 |
Correlations among needle parameters
An, Ap, Avas, D1, and D2 were closely correlated in control needles as well as in needles 227 DAG (Fig. 3 - left panel). In contrast, Fn was not correlated with other parameters in control needles or needles 227 DAG. Correlations among the studied needle parameters 411 DAG were less pronounced than in control needles and needles 227 DAG (Fig. 3 - left panel). Only six correlations were observed in needles 411 DAG and, of these, D1 was the most correlated needle parameter (Fig. 3 - left panel).
Fig. 3 - Correlation matrices (Pearson’s product-moment correlation) among needle parameters for control trees (upper panels), trees 227 days after girdling (DAG - middle panels) and trees 411 DAG (lower panels). Pearson’s correlation coefficient and significance level: black box (r≥0.6; p<0.05); white box (r<0.6; p>0.05);
Correlations between Ln, LMA, ND, and PLA were relatively weak for both control and girdled trees (Fig. 3 - right panel). The only exception was a correlation between Ln and PLA in control needles and needles 411 DAG, and a correlation between LMA and ND in needles 227 DAG. In addition, DM was correlated with Ln and PLA in control needles and needles 411 DAG (Fig. 3 - right panel). Although DM was used for the calculation of LMA and ND, they were not correlated.
Discussion
In this study, we observed several changes in needle structure in response to stem girdling 227 and 411 DAG, similarly to several previous investigations which measured physiological changes after stem girdling ([21], [28], [32], [19]). While the morphological and anatomical structures of needles 227 DAG were very similar to those of the control trees (the only marked difference was an increase of 9% in LMA, that is, the first hypothesis was not confirmed), seven of the eleven studied needle parameters were significantly different 411 DAG (in part, supporting our second hypothesis). The increase in LMA 227 DAG could be partly explained by a 5% increase in NSC in needles 227 DAG ([11]). LMA has previously been proposed as a useful indirect proxy for studying changes in carbohydrate concentrations ([5], [8], [15]). However, the increase in LMA in needles 227 DAG was higher than observed increases in NSC. This suggests that other mechanisms such as the accumulation of other NSCs or structural carbohydrates (i.e., an increase in the proportional representation of the cell wall) also contributed to the increase in LMA. Prolonged stem-girdling (411 DAG) decreased LMA and the concentration of insoluble NSC (starch) to the levels found in control needles. The initial increase in LMA after girdling and its subsequent decline was also found in Pinus canariensis C.Sm. seedlings ([15]) and Pinus ponderosa Dougal ex C. Lawson trees ([8]).
It has been reported that the reduction in stomatal conductance due to girdling results in lower transpiration, and therefore leads to a more favorable leaf water status ([34]). As cell expansion and division are positively affected by higher water potentials ([3]), an increase in the number and/or dimensions of cells should be expected. However, no increase in needle structural parameters was observed in any of the girdled trees, indicating declining water availability during needle development. The likely reason for the decrease in the leaf water potential of girdled trees is that as the roots are gradually depleted of their carbohydrate reserves ([33]), their surface area and absorptive capacity decrease, resulting in a subsequent increase in needle water shortage and eventual tree death ([22], [26]). This could be the reason why needles 411 DAG were smaller than control needles (supporting our second hypothesis). Moreover, needles 411 DAG had a smaller xylem area and a lower number of tracheids ([11]). Our results also correspond with a previous study ([1]) in which poorer leaf development and smaller leaf size were observed for three deciduous species in the second season following stem girdling. We should note that several other factors (site conditions, climate, tree age, nutritional status, and tree health) also play an important role in needle development along with carbohydrate concentration and water status. For example, Scots pine needles growing on dunes with poor water availability had An values similar to those of trees 411 DAG ([16]). In the present study, the mean values of D1 (0.56 mm) and D2 (0.91 mm) for control trees were at the lower end or below the range reported for adult Scots pine trees (0.4-0.72 mm and 1.13-1.32 mm, respectively - [14], [17], [16]). Interestingly, the reactions of D1 and D2 in response to girdling differed, as only D1 was observed to have decreased in needles 411 DAG. It seems that needles 411 DAG still had a sufficient water supply during the early stages of needle development (i.e., in May 2014, the stem sap flow rate in trees 411 DAG was 59 ± 10% of that of control trees). However, water was less available for xylem and phloem formation later in the season, leading to smaller values of D1 (i.e., the transpiration rate of trees 411 DAG decreased to 31 ± 9% of that of control trees during the last two weeks in June 2014). This uneven development of D1 and D2 after stem girdling was also the reason why needles 411 DAG were flatter in form than in the control trees. The idea of needle growth being restricted by an insufficient supply of water is also supported by the observed increases in ND in needles 411 DAG, as ND is a foliar characteristic that generally increases in response to water limitation ([23]). Nevertheless, the development of needle water potential after stem girdling should be investigated in future studies to demonstrate its influence on needle structural changes.
Regulatory mechanisms that determine needle size, and by extension needle form, are vital in that they allow plants to adapt to environmental conditions. It has been shown that anatomical changes in needles, from individuals to canopy level, are complex in nature ([10], [11]). These features are likely regulated by both endogenous and environmental factors ([2]). Complexity in growth patterns was also observed in our study, as several needle parameters were closely inter-correlated in control trees and in trees 227 DAG. In contrast, a smaller number of correlations that achieved the required level of significance were observed in needles 411 DAG (partially supporting our third hypothesis). It was also observed that the structural development determining Fn, ND, and LMA appeared to have driving factors that were independent of the other studied needle parameters, as correlations with other parameters were weak in all treatments. This suggests that gene expression in the needles of control and girdled trees could provide further insights into the regulatory mechanisms that determine the size and structural form of needles.
Acknowledgements
This work was funded by Czech project MSMT COST LD 13017 under the framework of the COST FP1106 network STReESS, by the Ministry of Education, Youth and Sports of the Czech Republic (Grant INTERTRANSFER LTT17033), and by the project “Indicators of Tree Vitality” (Grant No. CZ.1.07/2.3.00/20.0265) co-financed by the European Social Fund and the state budget of the Czech Republic.
Conflict of Interest
The authors declare that they have no conflicts of interest.
References
Gscholar
Authors’ Info
Authors’ Affiliation
Roman Plichta
Jirí Foit
Václav Cermák
Josef Urban
Mendel University in Brno, Faculty of Forestry and Wood Technology, Zemedelská 3, Brno, 61300 (Czech Republic)
Siberian Federal University, Krasnoyarsk (Russia)
Corresponding author
Paper Info
Citation
Gebauer R, Plichta R, Foit J, Cermák V, Urban J (2018). Long-term effects of stem girdling on needle structure in Scots pine. iForest 11: 476-481. - doi: 10.3832/ifor2648-011
Academic Editor
Silvano Fares
Paper history
Received: Oct 10, 2017
Accepted: Apr 05, 2018
First online: Jul 02, 2018
Publication Date: Aug 31, 2018
Publication Time: 2.93 months
Copyright Information
© SISEF - The Italian Society of Silviculture and Forest Ecology 2018
Open Access
This article is distributed under the terms of the Creative Commons Attribution-Non Commercial 4.0 International (https://creativecommons.org/licenses/by-nc/4.0/), which permits unrestricted use, distribution, and reproduction in any medium, provided you give appropriate credit to the original author(s) and the source, provide a link to the Creative Commons license, and indicate if changes were made.
Web Metrics
Breakdown by View Type
Article Usage
Total Article Views: 44937
(from publication date up to now)
Breakdown by View Type
HTML Page Views: 38935
Abstract Page Views: 2047
PDF Downloads: 3041
Citation/Reference Downloads: 8
XML Downloads: 906
Web Metrics
Days since publication: 2558
Overall contacts: 44937
Avg. contacts per week: 122.97
Article Citations
Article citations are based on data periodically collected from the Clarivate Web of Science web site
(last update: Mar 2025)
(No citations were found up to date. Please come back later)
Publication Metrics
by Dimensions ©
Articles citing this article
List of the papers citing this article based on CrossRef Cited-by.
Related Contents
iForest Similar Articles
Research Articles
Auxin (IAA) and soluble carbohydrate seasonal dynamics monitored during xylogenesis and phloemogenesis in Scots pine
vol. 11, pp. 553-562 (online: 01 September 2018)
Research Articles
Is it needed to integrate mixture degree in Stand Density Management Diagram (SDMD)?
vol. 16, pp. 274-281 (online: 28 October 2023)
Technical Notes
The feasibility of implementing cross-border land-use management strategies: a report from three Upper Silesian Euroregions
vol. 7, pp. 396-402 (online: 19 May 2014)
Research Articles
Vertical position of dry mass and elemental concentrations in Pinus sylvestris L. canopy under the different ash-nitrogen treatments
vol. 8, pp. 838-845 (online: 25 March 2015)
Research Articles
Use of terrestrial laser scanning to evaluate the spatial distribution of soil disturbance by skidding operations
vol. 8, pp. 386-393 (online: 08 October 2014)
Research Articles
Leaf morphology of progenies in Q. suber, Q. ilex, and their hybrids using multivariate and geometric morphometric analysis
vol. 11, pp. 90-98 (online: 31 January 2018)
Research Articles
Forest litter as the mulch improving growth and ectomycorrhizal diversity of bare-root Scots pine (Pinus sylvestris) seedlings
vol. 8, pp. 394-400 (online: 20 August 2014)
Research Articles
Measured and modelled source water δ18O based on tree-ring cellulose of larch and pine trees from the permafrost zone
vol. 13, pp. 224-229 (online: 19 June 2020)
Research Articles
Sap flow, leaf-level gas exchange and spectral responses to drought in Pinus sylvestris, Pinus pinea and Pinus halepensis
vol. 10, pp. 204-214 (online: 01 November 2016)
Review Papers
Indicators of drought effects in Pinus sylvestris: genetic analyses to corroborate the results of empirical methods
vol. 3, pp. 89-91 (online: 15 July 2010)
iForest Database Search
Search By Author
Search By Keyword
Google Scholar Search
Citing Articles
Search By Author
Search By Keywords
PubMed Search
Search By Author
Search By Keyword