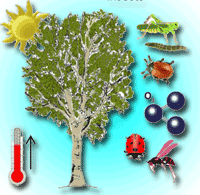
Leaf volatile isoprenoids: an important defensive armament in forest tree species
iForest - Biogeosciences and Forestry, Volume 5, Issue 1, Pages 13-17 (2012)
doi: https://doi.org/10.3832/ifor0607-009
Published: Feb 14, 2012 - Copyright © 2012 SISEF
Review Papers
Abstract
Current knowledge on the ecological impact of the emission of volatile isoprenoids by plants is reviewed. This trait is common to many terrestrial species but is scattered across different taxonomic groups; it appears to be related to other ecological traits rather than to phylogenetic relationships. Plants invest high resources to produce volatile isoprenoids, which are likely to play multiple roles in the defence against biotic and abiotic stressors. We describe how constitutive and induced volatile isoprenoids may directly or indirectly defend plants, and briefly address how indirect defence may involve communication with other trophic levels beyond the simple plant-herbivory interaction. It is discussed that, as metabolically costly defensive mechanisms are only activated after attacks, induced volatile isoprenoids may also prime other biochemical pathways that are involved in stress resistance responses. It is also surmised that attacked plants may also use volatiles as an airborne communication to signal the attack to other leaves or other plant organs, or even other plants, eliciting defence responses.
Keywords
Isoprene, Monoterpenes, Plant communication, Plant defence, Stress physiology
Introduction
Isoprenoids are a group of secondary metabolites produced by plants ([44]). The isoprenoid common precursor, isopentenyl pyrophosphate (IPP) may be enzymatically transformed into isoprene (the most abundant volatile isoprenoids), or may polymerize with its isoform dymetylallyl pyrophosphate (DMAPP). In the latter case, a range of volatile, semi-volatile, or non-volatile isoprenoids is formed, depending on the molecular weight and on the solubility of these molecules. Volatile isoprenoids (VIPs) are isoprene (C5) and monoterpenes (C10), which are made by the same chloroplast-based photosynthesis-dependent pathway (the MEP pathway, named from the 2-C-methyl-D-erythritol 4-phosphate intermediate), and homoterpenes (C11 and C16) and sesquiterpenes (C15), which are made in the cytosol from the classic mevalonate (MEV) pathway ([23], [40]).
This paper will review current understanding on the evolution and ecological roles of volatile isoprenoids, whereas non-volatile isoprenoids, which are known to also have important functions (e.g., the antioxidant function of xanthophylls and carotenoids, see [7]), will not be treated here.
VIPs are among the most well known Biogenic Volatile Organic Compounds (BVOCs). Because of their abundance and reactivity, once emitted in the atmosphere volatile isoprenoids play a crucial role in the interaction between biosphere and atmosphere, e.g., entering cycles of ozone and particle formation. This topic has been recently reviewed in great detail by Fowler et al. ([10]): we shall focus here on the other multiple ecological roles of volatile isoprenoids, as they have emerged from recent studies. We will mostly examine current knowledge about isoprene and monoterpenes, as forest tree species appear to produce and emit these compounds in large rates compared to other plant species, and their importance in protection against abiotic and biotic stresses is now emerging ([24], [8]).
Volatile isoprenoids can be emitted by different plant organs. Large and life-long emissions are found in photosynthetic organs (leaves and stems), but periodically large emissions can be detected from flowers ([21]) and roots ([38]). Emissions of constitutive blends of monoterpenes, homoterpenes, and sesquiterpenes by flowers are ontogenetically programmed and have an important ecological role in the communication between plants and their hosts, mainly pollinators for cross-fertilization ([17]). These aspects will not be treated here, being the main focus of this paper on foliar emissions of volatile isoprenoids.
Foliar volatile isoprenoids may accumulate in small temporary pools in the leaf mesophyll, or in large pools in specialized organs, e.g., resin ducts (conifers), or oil glands (Lamiaceae). In both cases, volatile isoprenoids may be constitutively emitted or the synthesis and emission may be stress-induced. In the following sections current knowledge on the evolution of constitutive isoprenoids will be reviewed, and ecological considerations about the functions of constitutive and stress-induced isoprenoids will be presented.
Evolution of costitutive isoprenoids
Emission of isoprenoids takes place in most groups of land plants: dicots, monocots, gymnosperms, pteridophytes and mosses ([19]). Nonetheless, distribution of emitting plants is scattered across the phylogeny of land plants, suggesting multiple gains and/or losses of such metabolites. Several hypotheses have been proposed by the scientific community regarding isoprene evolution: the key enzyme isoprene synthase (IspS) may either have evolved many times ([15]), or have been lost many times ([14]). More recently, Lerdau & Gray ([22]) suggested that monoterpene synthases forming the same products have evolved independently in widely separated groups (conifers and angiosperms).
Sharkey et al. ([35]) explained that, in angiosperms, isoprene synthases form a monophyletic group, but it is not yet possible to assess whether this means evolution from a common ancestral gene or convergence based on function. According to Affek & Yakir ([1]), isoprene is a strong antioxidant, and represents a primitive protection mode against singlet oxygen that evolved later into more dedicated radical scavengers. Vickers et al. ([44]) also seem to support the idea that isoprene is a primitive trait, and put forward the interesting speculation that isoprene evolved when plants became terrestrial, in order to help cope with high levels of oxygen and strong oxidative stress, which were non-existent in the oxygen-deprived aquatic medium.
Available inventories (e.g., [19]) may suggest that the emission of volatile compounds varies between ecologically different plants: isoprene emission characterises mostly hygrophylic trees (poplars, willows, pedunculate oak) and aquatic plants (common reed), whereas monoterpene emitters are mostly ecologically xerophylic. There is no definite evidence yet about such a correlation between the emission trait and the ecological classification of plants. However, one of the first experiments linking taxonomy and functions seems to indicate that the isoprene emission trait is significantly more widespread in hygrophylic tree species of the Italian flora (F. Loreto et al., unpublished).
Indeed, while chemotypes are distinguishable in plant species that store isoprenoids in large pools, e.g., conifers ([13], [33]), in large emitters that do not have storage pools there is apparently no relationship between isoprenoids emission and phylogeny. Genera of the same family, or species within the same genus (e.g., Quercus), may have different emission patterns, including both isoprene and monoterpene-emitting species, as well as non- or low-emitters ([26]). In Quercus suber (cork oak), - the only species not storing isoprenoids in which the emission of these volatile compounds has been studied at the intraspecific level ([37], [29]) -, different individuals emit different blends of monoterpenes. It may be suggested that these differences are not only caused by adaptation to the environment, but might rather be associated with past genetic isolation of cork oak populations, whose gene pools have been dated to originate several million years before present ([30]). For this reason, Loreto et al. ([29]) hypothesised that the emission profiles can be used as markers of intraspecific diversity, thus helping understand patterns of geographic variations associated with adaptation to the environment. Interestingly, however, the high emission of limonene by Portuguese cork oak populations has been proposed to occur because the trait is associated to high cork yield ([29]). Further studies are needed to unravel the genetic ground of this association between an agriculturally suitable trait and the pattern of isoprenoid formation.
Volatile isoprenoids and abiotic stresses
Almost all abiotic stress factors are able to affect isoprenoid biosynthesis and emission. Since abiotic stresses inhibit photosynthesis without exception, and since volatile isoprenoids are mainly formed by carbon directly derived from photosynthetic carbon metabolism, a concurrent negative effect of abiotic stresses on photosynthesis and isoprenoids emission would be expected. However, abiotic stresses generally stimulate biosynthesis and emission of constitutive isoprenoids (Fig. 1a). This is due to simultaneous: (i) elicitation of gene expression and increase of transcript levels of the genes involved in volatile isoprenoid biosynthesis; (ii) increasing contribution of carbon coming from the catabolism of photosynthates into the isoprenoid biochemical pathway; (iii) absence of stomatal closure limitation to emission of volatile isoprenoids ([24]).
Fig. 1 - Different roles of volatile isoprenoids (VIPs) in protecting plants from abiotic and biotic stress factors. (a) . Constitutive VIPs have a protective action against oxidant factors (e.g., ozone), high temperature and other environmental constraints. (b) Biotic stresses. Constitutive VIPs repel dangerous herbivores, thus acting as direct defence mechanism. On the other hand, some insects have learnt to use VIPs to locate the host plant, a remarkable example of co-evolution. (c) Biotic stresses. The attack by herbivorous insects induces emission of VIPs that attract natural enemies (e.g., carnivorous insects) as an indirect defence mechanism of the host plant. (d) Biotic stresses and plant communication. Induced emission of VIPs not only attract natural enemies of herbivorous insects but also help attacked plants communicate to neighbours the presence of risk.
Under environmental constraints that inhibit photosynthesis, the carbon released as volatile isoprenoids often becomes larger than the photosynthetic carbon uptake, and the foliar carbon budget becomes negative ([5]). When this occurs at the forest ecosystem level, the amount of carbon fixed by vegetation is reduced, thus strongly influencing both net ecosystem (NEP) and biome (NBP) productivity ([20]).
The search for the ecological reason and physiological mechanism(s) behind the observed elicitation of isoprenoid production (especially isoprene) under abiotic stress conditions (especially high temperatures and high oxidative conditions) has led to significant research in the past two decades (reviewed by [24]).
Ecologically, volatile isoprenoids are expected to increase fitness of high-emitting plants, thus accounting for the large metabolic costs of their stress-enhanced biosynthesis. In brief, the two theories that have received prominent experimental support are that: (a) volatile isoprenoids strengthen cellular membranes, thus maintaining the integrity of the thylakoid-embedded photosynthetic apparatus (first postulated by [36]); (b) volatile isoprenoids have a more generic antioxidant action, deactivating reactive oxygen species around and inside leaves, thus indirectly reducing oxidation of membrane structures and macromolecules (first proposed by [25]). The two effects of isoprenoids are possibly combined, as explained recently by Loreto & Schnitzler ([24]) and Velikova et al. ([43]). Yet, experiments with transgenic plants have brought important confirmation that stabilization of membrane integrity may be the primary mechanism that allows maintenance of photosynthetic rates and reduces reactive oxygen species production under stress conditions ([42]). Behnke et al. ([3]) have recently demonstrated that repressing isoprene biosynthesis in high-emitting poplars might provide more carbon for photosynthesis and growth in stress-free environments, although not to the expected extent. However, non-emitting poplars may become more susceptible to herbivores and abiotic stresses. The experiment of Behnke et al. ([3]) suggests that isoprene emission may not be essential for plant survival in temperate habitats, but does not rule out a more significant role of isoprene under stress conditions.
Volatile isoprenoids and biotic interactions: direct and indirect defence
The use of volatile molecules for communication of plants with other organisms is a well-known and fascinating field of study. We have earlier mentioned the use of floral cues to attract pollinators. In this and other insect-plant mutualisms, partners involved play different roles. The sessile partner (the plant) needs to be easily localised and offers rewards (mostly food) to the mobile partner (the insect), who offers a service but also makes its choice in visiting or not a particular individual. This implies that plants have evolved specific traits to be invested in mutualism, whereas insects have not. In fact, the behavioural repertoire utilised by mutualist insects does not differ from that of their relatives not involved in mutualistic associations. Such an asymmetry is particularly obvious in more generalised insect-plant mutualisms ([6]).
Plant volatiles are not always useful to the emitting plants (Fig. 1b). Herbivores have evolved the capacity to use volatiles as cues to localize their host. This is typical of constitutive volatiles whose blend is species-specific and is not affected qualitatively by environmental conditions. Numerous examples of such a co-evolution exist in nature, and some of them also apply to forest trees. In poplars, whose adult leaves only emit isoprene at very high rates, the beetle Chrysomela (syn.: Melasoma) populi has learnt that expanding leaves also emit monoterpenes and use this cue to orientate and land on a more rewarding food source ([5]). Plants also co-evolve, though at a likely slower rate than their animal hosts, and the strategies to defend themselves against herbivores again make use of volatile compounds, including induced isoprenoids. Metabolic changes in plants attacked by herbivores lead to the production of secondary compounds, often named Herbivore-Induced Plant Volatiles (HIPVs), including several volatile isoprenoids that can directly deter herbivores (direct defence), or attract herbivores’ parasitoids or predators, thus inducing an indirect induced defence resulting from the plant interaction with insects of the third trophic level, i.e., carnivores (Fig. 1c - [9], [31], [8]). There is again a wealth of examples about direct and indirect defence involving volatile isoprenoids. Volatile isoprenoids contained in the resins of conifers are particularly abundant in young trees where they successfully deter herbivores from feeding ([27]) and may even help rapidly sealing mechanical wounds ([34]). Although indirect defence has been mostly studied in herbaceous crops, some interactions involving forest trees were also investigated, as reviewed by Holopainen et al. ([18]).
Attention has been recently given to even more sophisticated interactions that also involve activation of protection mechanisms through volatile isoprenoid-induced priming or signalling. This is addressed in the next section.
Volatile isoprenoids and biotic interactions: priming and signalling
Defence mechanisms imply high metabolic costs ([39]). For this reason plants might have evolved mechanisms to be triggered only as consequence of herbivore attack; this explains why only induced volatile isoprenoids are generally active in the indirect defence. Such strategy may also require priming of biochemical mechanisms (mainly activation of stress-induced proteins) preparing plant tissues to react against upcoming attack in a less costly though effective way (e.g., through metabolites with antioxidant and antinutritional properties - [16]). Priming does not confer resistance per se, but makes plants ready for induced resistance when an attack occurs. In this way, it is less costly than a fully implemented defence response ([12]). Induced defence strategies may also require signalling - to other leaves or plant organs (e.g., belowground/aboveground), or even other plants - that the infection/infestation has occurred ([2]). As for the other defence strategies briefly reviewed above, they may limit the diffusion of herbivore animals, which can quickly move within the plant, or among plants, and cause damages to different organs.
A remarkable example of a coordinated response involving priming and signalling is at the basis of the so called Systemic Induced Resistance ([16]). Systemic Induced Resistance can be activated in distal organs not yet affected by the attack by two different mechanisms, both supported by experimental evidence: (i) a systemic transport of (already existing) defensive metabolites; and (ii) a de novo expression of resistance mechanism activated by translocated signals from the stressed tissue to distal undamaged tissues. Translocation of signals was traditionally assumed to take place through the vascular system. However, it is now clear that airborne translocation (external transmission of signals by volatiles emitted from the damaged tissues) is also possible and can trigger defensive mechanisms in remote organs both in the same individual and in neighbours (Fig. 1d - [16]). According to Heil & Ton ([16]) there are several reasons explaining why airborne signalling may provide plants with important benefits. First, airborne transportation is a cue that elicits defence responses in those plant parts where resistance is mostly required. Second, signal transmission in the air is faster than the vascular one. Third, airborne and vascular defence signals can act in synergy. Fourth, volatile-mediated signalling works most efficiently over relatively short distances. In this way, the probability that the leaf nearest to an attacked one belongs to the same plant is fairly high.
Volatile molecules are the best candidates as within- and between-plant signals indicating the presence of herbivores: this info-chemical communication ([11]) implies the aerial transfer of information from a herbivore-attacked plant (the emitter) to close growing plants (the listening receivers, or eavesdropping plants). By eavesdropping HIPVs released from infested neighbors, phenotypes may be tailored to increase fitness. Selection does not favour plants passing important information to competitors ([2]). Therefore, eavesdroppers may take advantage of within-plant signalling mechanisms of infected/infested neighbouring HIPVs emitters ([16]), thus increasing their own fitness.
In fact, within-plant communication may be more effective than plant-to-plant communication in nature. Distance is a crucial parameter in plant-to-plant signalling, and positive reactions are restricted to close surroundings of the emitters. The distance over which airborne cues can affect other plants strongly depends on wind speed, air humidity, and temperature, but in general it appears to be very short ([16]), especially when emitted volatiles are very reactive, as is the case for most volatile isoprenoids. These compounds also rapidly react with atmospheric pollutants, especially ozone and NOx, rapidly disappearing in polluted environments ([32], [4]).
The high reactivity of volatile isoprenoids also makes it possible other, more indirect, priming mechanisms. By rapidly reacting with NO ([41]) and H2O2 ([25]), isoprene (and perhaps also other volatile isoprenoids) may indirectly quench the two above compounds that are responsible for signalling hypersensitive responses at cellular level ([45]). The low hypersensitive response may explain the low damage observed in ozonated leaves in an isoprene-rich environment ([28]). Finally, preliminary results indicate that heavy emitters of volatile isoprenoids may have higher - yet not pathological - levels of these signalling molecules (especially H2O2) when grown under non-stressed conditions. This fine metabolic adjustment may indicate an additional form of priming able to immunize plants against forthcoming stresses. Further studies are needed to throw light on the above mechanisms, whose impacts on the physiology of stress resistance may be very relevant.
In summary, while recent developments indicate that volatile isoprenoids may not be essential for plant survival in unstressed conditions, an outstanding body of information is now available confirming the multiple roles of these molecules in plant protection against stresses. As environmental and man-made changes (including air pollution and land-use changes) largely affect the capacity to produce and emit biogenic volatile isoprenoids, they may also disturb the defensive and communication performances of plants. The opportunity of producing genetically transformed plants that do not emit volatile compounds (“mute” emitters) and that do not receive these volatile signals (“deaf” plants) represents a extraordinary tool for assessing the importance in nature of volatile isoprenoid signalling and whether they may enhance the plant fitness both in natural communities ([2]) and in response to current and future climate change.
References
CrossRef | Gscholar
CrossRef | Gscholar
CrossRef | Gscholar
Authors’ Info
Authors’ Affiliation
F Loreto
Istituto per la Protezione delle Piante (IPP), Consiglio Nazionale delle Ricerche (CNR), v. Madonna del Piano 10, I-50019 Firenze (Italy)
Corresponding author
Paper Info
Citation
Fineschi S, Loreto F (2012). Leaf volatile isoprenoids: an important defensive armament in forest tree species. iForest 5: 13-17. - doi: 10.3832/ifor0607-009
Academic Editor
Gabriele Bucci
Paper history
Received: Nov 30, 2011
Accepted: Feb 07, 2012
First online: Feb 14, 2012
Publication Date: Feb 27, 2012
Publication Time: 0.23 months
Copyright Information
© SISEF - The Italian Society of Silviculture and Forest Ecology 2012
Open Access
This article is distributed under the terms of the Creative Commons Attribution-Non Commercial 4.0 International (https://creativecommons.org/licenses/by-nc/4.0/), which permits unrestricted use, distribution, and reproduction in any medium, provided you give appropriate credit to the original author(s) and the source, provide a link to the Creative Commons license, and indicate if changes were made.
Web Metrics
Breakdown by View Type
Article Usage
Total Article Views: 67508
(from publication date up to now)
Breakdown by View Type
HTML Page Views: 56419
Abstract Page Views: 3344
PDF Downloads: 5995
Citation/Reference Downloads: 66
XML Downloads: 1684
Web Metrics
Days since publication: 4894
Overall contacts: 67508
Avg. contacts per week: 96.56
Article Citations
Article citations are based on data periodically collected from the Clarivate Web of Science web site
(last update: Mar 2025)
Total number of cites (since 2012): 29
Average cites per year: 2.07
Publication Metrics
by Dimensions ©
Articles citing this article
List of the papers citing this article based on CrossRef Cited-by.
Related Contents
iForest Similar Articles
Research Articles
A new approach to ozone plant fumigation: The Web-O3-Fumigation. Isoprene response to a gradient of ozone stress in leaves of Quercus pubescens
vol. 1, pp. 22-26 (online: 28 February 2008)
Research Articles
The use of branch enclosures to assess direct and indirect effects of elevated CO2 on photosynthesis, respiration and isoprene emission of Populus alba leaves
vol. 1, pp. 49-54 (online: 28 February 2008)
Research Articles
Spruce budworm biological and nutritional performance responses to varying levels of monoterpenes
vol. 6, pp. 310-314 (online: 16 July 2013)
Research Articles
Links between phenology and ecophysiology in a European beech forest
vol. 8, pp. 438-447 (online: 15 December 2014)
Research Articles
Oak sprouts grow better than seedlings under drought stress
vol. 9, pp. 529-535 (online: 17 March 2016)
Research Articles
Effect of drought stress on some growth, morphological, physiological, and biochemical parameters of two different populations of Quercus brantii
vol. 11, pp. 212-220 (online: 01 March 2018)
Research Articles
Nutrient uptake, allocation and biochemical changes in two Chinese fir cuttings under heterogeneous phosphorus supply
vol. 11, pp. 411-417 (online: 05 June 2018)
Research Articles
Use of δ13C as water stress indicator and potential silvicultural decision support tool in Pinus radiata stand management in South Africa
vol. 12, pp. 51-60 (online: 24 January 2019)
Research Articles
Tracing the acclimation of European beech (Fagus sylvatica L.) populations to climatic stress by analyzing the antioxidant system
vol. 14, pp. 95-103 (online: 01 March 2021)
Research Articles
Adaptability of Indocalamus decorus to climate change based on physiological and biochemical responses to elevated carbon dioxide and ozone
vol. 9, pp. 311-317 (online: 22 October 2015)
iForest Database Search
Search By Author
Search By Keyword
Google Scholar Search
Citing Articles
Search By Author
Search By Keywords
PubMed Search
Search By Author
Search By Keyword